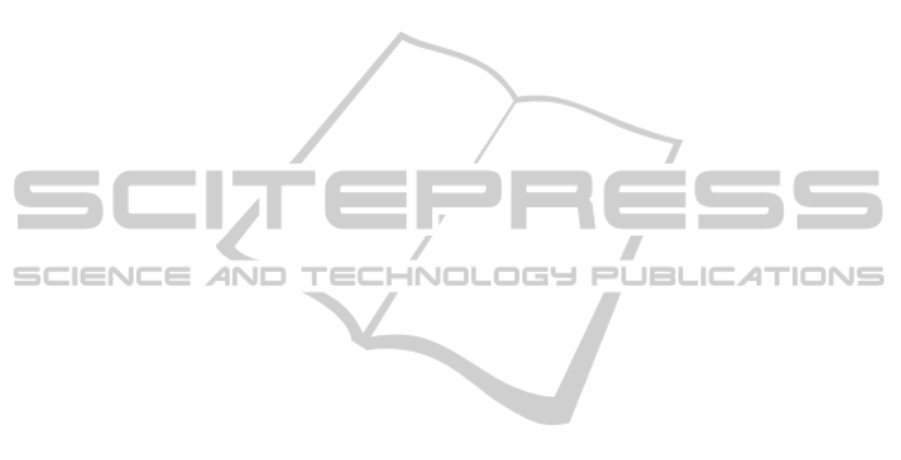
4 EDUCATIONAL IMPACTS
One key mission of universities is to educate the
future generation in their chosen career fields. An
equal and commensurate mission of universities is
the advancement of new knowledge. Faculty are
involved in research on the cutting-edge and
students are learning the ropes so they can graduate
with the most up-to-date understanding of the world.
So universities are natural places to investigate the
adoption of new technologies, and in this case, the
transformation of transportation from fossil fuels to
electricity. It is a huge effort that spans many
engineering fields, but also intersects with business
majors, supply chain, and social sciences in many
ways.
With our relatively new effort studying EV usage
patterns we have already had many chances to
intersect with students and develop further
understanding about EV usage. For example the
author teaches a solar device technology class with
thematic semester projects required of all students.
Recently one of these project assignments was
aimed at having the students design parking-lot
solar-arrays with the added feature of stationary
battery storage for storm resiliency. Also, one
engineering capstone design team is underway
examining electricity usage by EV fleet vehicles
being operated by the university – with the aim of
providing advice about recharging strategies and
understanding total cost of ownership for these new
vehicles.
EV data and energy strategies are also useful for
outreach in a variety of ways. For example the
author has given several presentations in the
“Energy Café” series organized by the Rutgers
Energy Institute. These are open to the public,
though mostly attract interested students.
In the future we expect students to be engaged in
research projects examining many facets of the
electrification of transportation in the region. For
example: Could EV’s be charged at the university’s
solar array during storm/grid failures and then used
for emergency delivery of power to critical facilities
in the region? This would build on our recent
probability model for guiding battery size for these
resilient power islands (Birnie 2014).
Also, could electric buses be used within the
sprawling university campus? What infrastructure,
performance and environmental impacts would
result (Rutgers maintains one of the largest bus
systems in the state of New Jersey).
Further, could “vehicle-to-grid” (V2G) systems
be fielded on or near campus? New variants of V2G
could be tested and evaluated within the context of a
large commuter population, both of students and for
faculty and staff. Again there are significant
infrastructure, logistics, and social changes that will
be required to allow for smooth operation of V2G
and other complicated energy systems in the future.
Already we are increasing our data gathering
capabilities and will be connecting these data with
driving habits, seasonal temperature variations, and
commuting routes. Our overall aim is to have
students involved in the data gathering, analysis, and
interpretation so that we can increase the impact for
regional transportation modification in the future.
5 INSTALLATION ISSUES
The transformation that we envision is hampered
significantly by the infrastructure needed to provide
power to growing numbers of commuters. The EV
charging hardware is only part of the story as
electric conduit may have to be laid and new circuits
added, depending on the location and anticipated
number of vehicles to be serviced. Up to this point
these infrastructure costs are quite a bit larger than
the value of the electricity that the vehicles receive.
Also, the EV equipment that we have installed so
far has been added with relatively little consideration
of the population of likely users and their charging
habits and how this impinges on the general
limitation on availability of parking. And, the
question of different usage patterns that will match
with Level 1, Level 2 or higher power rates has not
been clarified.
The best strategy will likely be to combine new
EV charging locations with new construction
projects and building renovations so that the
rewiring and new hardware can be made as cost-
effective as possible. And, there may be new ways
of co-funding for charging units that will be used
partly by the university fleet and partly by the
student/faculty/staff private vehicles. This is a wide-
open discussion that is evolving rapidly on campus
as we move this initiative forward.
6 POLICY CONNECTIONS
Our studies of electric transportation integrate the
technological (hardware and algorithms) with the
social (attitudes and behaviour patterns). In many
cases these combined socio-technological changes
will be assisted by policy choices that we make
VEHITS2015-InternationalConferenceonVehicleTechnologyandIntelligentTransportSystems
96