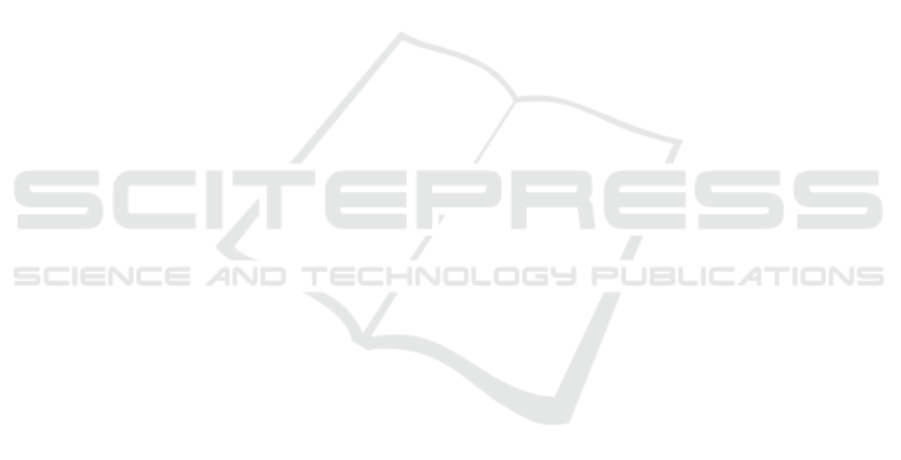
ticular, whether it is feasible to measure quantitative
parameters, particularly tidal volume and minute ven-
tilation, as well as breathing phase timing, under ”dy-
namic” conditions).
In our previous work, we confirmed that a cali-
bration procedure is needed (particularly for various
body positions) to measure quantitative parameters
with accuracy comparable to the reference. However,
we provided those results and consideration for static,
”clear” conditions. They were free of possibly dis-
torting maneuvers, such as irregular, shallow, inter-
mittent breathing; quick movements; changes in elec-
trode attachment (pressure of contact with the skin);
or non-breathing-associated changes in the volume of
the thorax, etc. These could influence the calibration
coefficient in a way that would only allow one to state
qualitative information about the depth of breathing.
One-lead ECG appears to provide results that do
not allow clinical investigation and diagnosis, but do
allow screening-like conclusions with greater com-
fort. Occurrences of the cardiac IP component may
lead to the concept of removing the classic ECG mea-
surement, however it seems, that cardiac IP compo-
nent is not fully visible in each participant. In our
opinion, if we are able to use the same electrodes to
measure impedance and ECG signal, it will be worth
having the redundant measurements for verification
purposes.
Removing separated ECG input for combined one
is prepared in order to improve the usability of Pneu-
monitor 3. It is also related with motion tracking. We
decided to limit ourselves to a single inertial unit (in-
cluding both accelerometer and gyroscope sensors)
to maintain comfort and accuracy of detecting basic
body positions and activity levels (Bouten et al., 1997;
Ermes et al., 2008).
5 CONCLUSIONS
Prototypes of two devices intended for cardiorespi-
ratory measurements in ambulatory conditions (with
motion tracking, including activity and body position
changes) were prepared and preliminarily evaluated.
The devices utilize impedance pneumography
to calculate respiratory parameters (tidal volume,
minute ventilation and respiratory rate) after calibra-
tion, ECG to record heart activity, and a combina-
tion of 3-axis accelerometer and gyroscope to track
motion. Pneumonitor 3 also includes pulse oximetry
providing saturation and pulse wave, and uses 5 elec-
trodes setting with 2 ECG electrodes combined with
receiving I P ones.
Pneumonitor 2 is designed for the environment
physiology analyses (registering ventilation and car-
diac functioning in subjects with obesity or nervous-
muscle-related illnesses) and sports medicine (for am-
bulatory diagnostics, monitoring training, and deter-
mining exercise capacity). Pneumonitor 3 is intended
mainly for sleep studies to monitor breathing disor-
ders and the treatment progress.
REFERENCES
Bouten, C. V., Sauren, A. A., Verduin, M., and Janssen, J.
(1997). Effects of placement and orientation of body-
fixed accelerometers on the assessment of energy ex-
penditure during walking. Med Biol Eng Comput,
35(1):50–56.
Collop, N. A., Anderson, W. M., Boehlecke, B., Claman,
D., Goldberg, R., Gottlieb, D., Hudgel, D., Sateia, M.,
and Schwab, R. (2007). Clinical guidelines for the
use of unattended portable monitors in the diagnosis
of obstructive sleep apnea in adult patients. J Clin
Sleep Med, 3(7):737–747.
Ermes, M., Parkka, J., Mantyjarvi, J., and Korhonen, I.
(2008). Detection of daily activities and sports with
wearable sensors in controlled and uncontrolled con-
ditions. IEEE T Inf Technol B, 12(1):20–26.
Grossman, P. and Taylor, E. W. (2007). Toward under-
standing respiratory sinus arrhythmia: relations to car-
diac vagal tone, evolution and biobehavioral func-
tions. Biol Psychol, 74(2):263–285.
Hoyer, D., Frasch, M., Eiselt, M., Hoyer, O., and Zwiener,
U. (2001). Validating phase relations between cardiac
and breathing cycles during sleep. IEEE Eng Med
Biol, 20(2):101–106.
McNicholas, W. (1997). Impact of sleep in respiratory fail-
ure. Eur Respir J, 10(4):920–933.
Miller, M. R., Crapo, R., Hankinson, J., Brusasco, V., Bur-
gos, F., Casaburi, R., Coates, A., Enright, P., van der
Grinten, C. M., Gustafsson, P., et al. (2005). General
considerations for lung function testing. Eur Respir J,
26(1):153–161.
Mlynczak, M., Niewiadomski, W., Zylinski, M., and Cy-
bulski, G. (2015). Assessment of calibration methods
on impedance pneumography accuracy. Biomed Eng-
Biomed Te, 61(6):587–593.
Mlynczak, M. C., Niewiadomski, W., Zylinski, M., and Cy-
bulski, G. P. (2014). Ambulatory impedance pneu-
mography device for quantitative monitoring of volu-
metric parameters in respiratory and cardiac applica-
tions. In Computing in Cardiology, pages 965–968.
Roebuck, A., Monasterio, V., Gederi, E., Osipov, M., Behar,
J., Malhotra, A., Penzel, T., and Clifford, G. (2013). A
review of signals used in sleep analysis. Physiol Meas,
35(1):R1.
Seppa, V. P., Hyttinen, J., Uitto, M., Chrapek, W., and Viik,
J. (2013). Novel electrode configuration for highly lin-
ear impedance pneumography. Biomed Eng-Biomed
Te, 58(1):35–38.
BIODEVICES 2017 - 10th International Conference on Biomedical Electronics and Devices
96