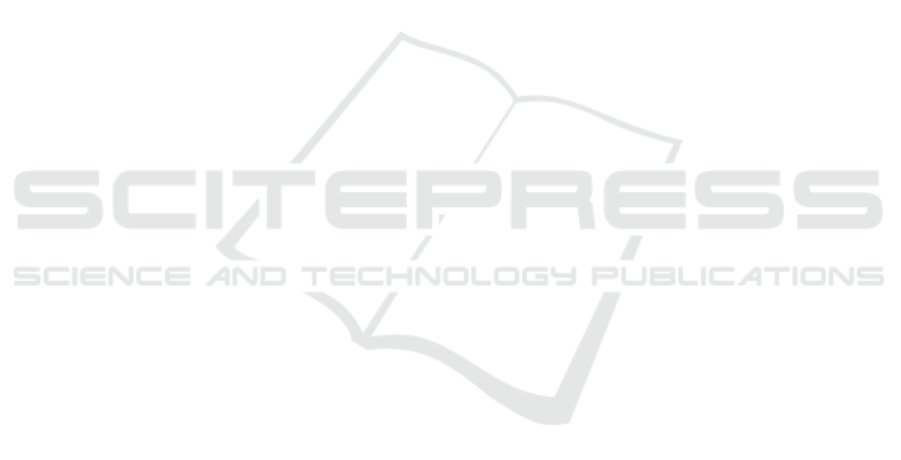
Koptyug, A., Rännar, L.-E., Bäckström, M. and Cronskär,
M. (2014). Additive Manufacturing for Medical and
Biomedical Applications: Advances and Challenges.
Materials Science Forum; 783-786, pp.1286-1291.
Koptyug, A., Rännar, L.-E., Bäckström, M. and
Surmenev, R. (2017). Additive manufacturing in
metal: advances generate new research challenges.
Nanotehnology: development and applications in XXI
century; 8(4), pp. 12-36.
Koptyug, A., Rännar, L.-E., Bäckström, M. and Shen, Z.
(2017). New metallurgy of Additive Manufacturing in
Metal: Experiences from the Material and Process
Development with Electron Beam Melting
Technology (EBM). Materials Science Forum; 879,
pp. 996-1001.
Koptyug, A., Bäckström, M. and Petrone, N. (2017).
Developing an Instrumented Physical Model of the
Human Head for Studying Concussion Mechanisms.
Proc. 22nd Congress of the International Society for
Skiing Safety, Innsbruck, April, 17 – 22 2017, p. 81.
Kuzmin Ski Technology AB (2017)
http://www.kuzmin.se/pgs/skiing_en.html
MakerBot Industries, LLC (2017)
https://www.makerbot.com/products/3d-printers/
Mills, D. (2015). Future Medicine: The Impact of 3D
Printing. J Nanomater Mol Nanotechnol 4:3.
MIPS AB, http://www.mipsprotection.com/
Murr, L,. Gaytan, S., Medina, F., Lopez, H., Martinez, E.,
Machado, B., Hernandez, H., Martinez, L., Lopez, M.,
Wiker, B. and Bracke, J. (2010). Next-generation
biomedical implants using additive manufacturing of
complex, cellular and functional mesh arrays”, Phil.
Trans. Roy. Soc. A; 368, pp. 1999-2032.
Niinomi, M. and Nakai, M. (2011). Titanium-Based
Biomaterials for Preventing Stress Shielding between
Implant Devices and Bone. Intl. J. Biomater.; 2011,
Article ID 836587.
Pallis, J. Materials in Paralympic Sports. Chapter 14, in:
Materials in Sports Equipment, Volume 1. Ed. Mike
Jenkins, Elsevier, 2003 - Technology & Engineering -
424 pages
Palousek, D., Rosicky, J., Koutny, D., Stoklasek, P. and
Navrat, T. (2014). Pilot study of the wrist orthosis
design process. Rapid Prototyping Journal; 20(1), pp.
27-32.
Pauly, S., Löber, L., Petters, R., Stoica, M., Scudino, S.,
Kühn, U., and Eckert J. (2013). Processing metallic
glasses by selective laser melting. Materials Today;
1(1-2), pp. 37-41.
Payne, T., Mitchell, S. and Bibb, R. (2013). Design of
human surrogates for the study of biomechanical
injury: a review. Critical Reviews in Biomedical
Engineering; 41 (1), pp. 51-89.
Payne, T., Mitchell, S., Halkon, B.; Bibb, R. and Waters,
M. (2015). Development of novel synthetic muscle
tissues for sports impact surrogates. J. Mechanical
Behavior of Biomedical Materials; 41, pp. 357-374.
Petrone, N., Tamburlin, L., Panizzolo, F. and Atzori, B.
(2010). Development of an instrumented
anthropomorphic dummy for the study of impacts and
falls in skiing. Procedia Engineering, 2(2), pp.2587-
2592.
Samaka, H. and Tarlochan, F. (2013). Finite Element (FE)
Human Head Models / Literature Review. Intern. J. of
Sci. Tech. Research, 2(7), pp. 17-21.
Sames, W., List, F., Pannala, S., Dehoff, R and Babu, S.
(2016). The metallurgy and processing science of
metal additive manufacturing. International Materials
Reviews; 6 (5), pp. 315-360.
Skoglund, P. (2915). Prosthetic socket in Titanium, Mid
Sweden University Master Thesis. Download
available at: https://www.diva-
portal.org/smash/get/diva2:901421/FULLTEXT01.pdf
Smith, T., Halstead, P., McCalley, E., Kebschull, S.,
Halstead, S. and Killeffer, J. (2015) Angular head
motion with and without head contact: implications for
brain injury. Sports Engineering, 18(3), pp. 165-175.
Special issue: Technology for Disability Sport. (2016).
Sports Engineering; 9(3); Eds. Koptyug, A., Burkett,
D. pp 139.
Stratasys Ltd. (2017) http://www.stratasys.com/3d-
printers
Sumner, D. (2015). Long-term implant fixation and stress-
shielding in total hip replacement. J Biomech.; 48(5),
pp. 797-800.
Surmenev, R., Surmeneva, M. and Ivanova, A. (2014).
Significance of calcium phosphate coatings for the
enhancement of new bone osteogenesis–A review.
Acta biomaterialia; 10 (2), pp. 557-579.
Surmeneva, M., Chudinova, E., Syrtanov, M., Koptyug, A.
and Surmenev, M. (2015). Investigation of the HA
film Deposited on the Porous Ti6Al4V Alloy Prepared
via Additive Manufacturing. IOP Conference Series:
Material Science and Engineering; 98, 012025.
Taha, Z., Hassan, M., Aris, M. and Anuar, Z. (2013).
Predicting brain acceleration during heading of soccer
ball. IOP Conf. Series: Materials Science and
Engineering, 50(1), 012023.
Tinnsten, M., Peter Carlsson, P., Ainegren, M., and
Sandberg, S. (2010). Arrangement for a roller ski and
a roller ski. European patent EP 2418006.
Zadpoor, A. and Malda, J. (2917). Additive Manufacturing
of Biomaterials, Tissues, and Organs. Annals of
Biomedical Engineering; 45(1), pp. 1–1.
Zhong, Y., Rännar, L.-E., Liu, L., Koptyug, A., Wikman,
S., Olsén, J., Cui, D. and Shen, Z. (2017) Additive
manufacturing of 316L stainless steel by electron
beam melting for nuclear fusion applications. Journal
of Nuclear Materials; 486, pp. 234-245.
Zhong, Y., Rännar, L.-E., Wikman, S., Koptyug, A., Liua,
L., Cui, D. and Shen, Z. (2017). Additive
manufacturing of ITER first wall panel parts by two
approaches: Selective laser melting and electron beam
melting. Fusion Engineering and Design; 116, pp. 24–
33.