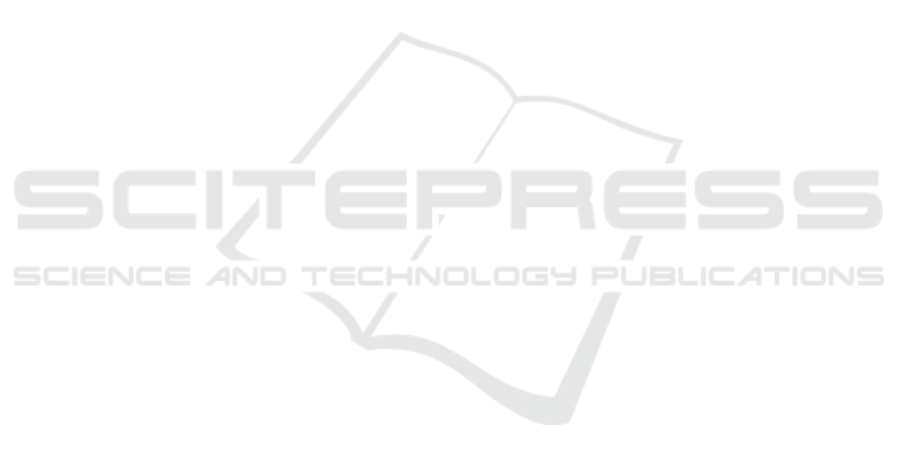
Nada, M, Muramoto, Y., Yokoyama, H., Ishibashi, T., and
S. Kodama, “InAlAs APD with high multiplied
responsivity-bandwidth product (MR-bandwidth
product) of 168 A/W.GHz for 25 Gbit/s high-speed
operations”, Electron. Lett., vol. 48, no. 7, pp. 397–399,
(2012).
Nada, M., Yoshimatsu,T., Muramoto, Y., Yokoyama, H.
and Matsuzaki, H., “Design and Performance of High-
Speed Avalanche Photodiodes for 100-Gb/s Systems
and Beyond,” IEEE/OSA Journal of Lightwave
Technology, vol. 33, no. 5, pp. 984-990, (2015).
Campbell, J. C., Demiguel, S., Ma, F., Beck, A., Guo, X.,
Wang, S., Zheng, X., Li, X., Beck, J. D., Kinch, M. A.,
Huntington, A., Coldren, L. A., Decobert, J. and
Tscherptner, N., “Recent Advances in Avalanche
Photodiodes,” IEEE J. of Sel. Topics in Quantum
Electronics, vol. 10, pp. 777-787, (2004).
Huang, J.S., Chang, H.S., Jan., Y.H., Ni, C.J., Chen, H.S.,
and Chou, E., ”Temperature dependence study of mesa-
type InGaAs/InAlAs avalanche photodiode
characteristics”, Adv. Optoelectron., no. 2084621, p.1-
5, (2017).
Laird, J.S., Hirao, T., Onoda, S., Ohyama, H., and T.
Kamiya, T., “Heavy-ion induced single-event transients
in high-speed InP-InGaAs avalanche photodiodes”,
IEEE Tran. Nuclear Sci., Vol. 50, No. 6, p.2225-2232,
(2003).
Alpert, A., “High-speed jitter testing of XFP transceivers”,
Viavi White Paper, (2015).
Tyagi, M.S., “Zener and avalanche breakdown in silicon
alloyed p-n junctions” Solid State Electron., vol. 11,
p.99-128, (1968).
Tan, L.L. J., Ong, D.S.G., Ng, J.S., Tan, C.H., Jone, S.K.,
Qian Y., and David, J.P.R., “Temperature dependence
of avalanche breakdown in InP and InAlAs”, IEEE J.
Quantum. Electron., vol.46, no.8, p.1153-1157, (2010).
”Generic reliability assurance requirements for
optoelectronic devices used in telecommunication
equipment”, Telcordia, GR-468-CORE, (2004).
Black, J.R., ”Electromigration failure modes in aluminum
metallization for semiconductor devices”, Proc. IEEE,
vol.57, no.9, p.1587-1594, (1969).
Huang, J.S., ”Temperature and current dependences of
reliabliity degradation of buried heterostrcture
semiconductor lasers”, IEEE Tran. Device Mater.
Reliab. Vo.5, no.1, p.150-154, (2005).
Huang, J.S., Nguyen, T., Hsin, W., Aeby, I., Ceballo, R.,
and Krogen, J., ”Reliability of etch-mesa buried
heterostructure semiconductor lasers”, IEEE Tran.
Device Mater. Reliab. Vo.5, no.4, p.665-674, (2005).
Ishimura, E., Yagyu, E., Nakaji, M., Ihara, S., Yoshiara, K.,
Aoyagi, T., Tokuda, Y., and Ishikawa, T., ”Degradation
mode analysis on highly reliable guardring-free planar
InAlAs avalanche photodiodes”, J. Lightwave Tech.,
vol. 25, no. 12, p.3686-3693, (2007).
Watanabe, I., Tsuji, M., Hayashi, M., Makita K., and
Taguchi, K., ”Reliability of Mesa-Structure InAlGaAs-
InAlAs superlattice avalanche photodiodes”, IEEE
Photonics Tech. Lett., Vol. 8, No. 6, p.824-826, (1996).
PHOTOPTICS 2018 - 6th International Conference on Photonics, Optics and Laser Technology
124