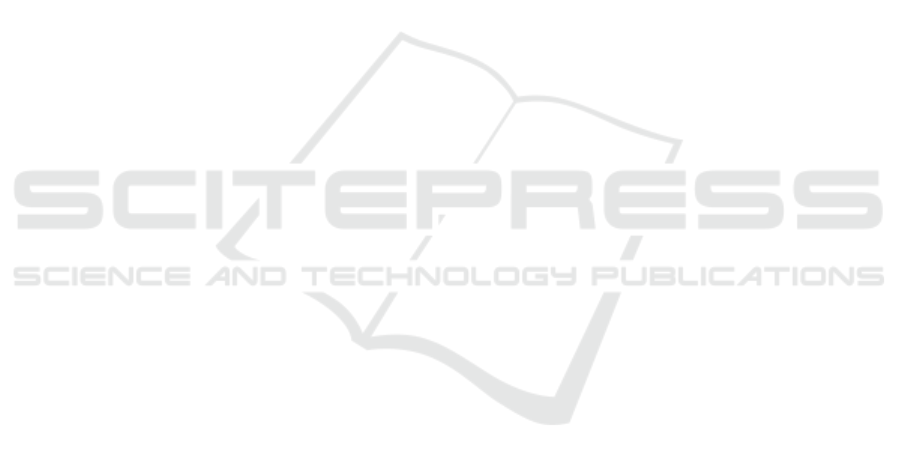
and sharp. The behaviour of a chalcogenide fiber
losses for different diameters was simulated by using
the FEM. Results obtained from the modelling study
also give an indication of the influence of the core size
in the fiber attenuations. The typical difference
between the loss of the 13 and 25 µm core diameter
fiber is only of 1 dB/m. This opens the possibility to
achieve a new chalcogenide fiber bundle with even
smaller core diameter and without substantial
increase in optical losses. It is then possible to include
a higher number of pixels (from 1200 to 3600) while
maintaining the bundle outer diameter small enough
to ensure mechanical flexibility, which will be the
focus of the future work.
REFERENCES
ARCoptix FT-IR Rocket data sheet, 2015. Available at:
http://www.arcoptix.com/pdf/Data%20Sheet%20-%20
Arcoptix%20FTIR%20Rocket.pdf (Accessed: 13
February 2017).
Berenger J.P., 1994. A Perfectly Matched Layer for the
Absorption of Electromagnetic Waves, Journal Of
Computational Physics 114, pp. 185-200.
Chenard, F., Alvarez, O., D. Gibson, D., Brandon Shaw, L.,
Sanghera, J., 2017. Mid-Infrared Imaging Fiber
Bundle, SPIE 10181, (2017).
COMSOL Multiphysics, 2017. Avilable at: https://www.
comsol.com/comsol-multiphysics (Accessed: 11 April
2017).
FEP Handbook. Available at: http://www.rjchase.com/
fep\_handbook.pdf, (Accessed: 19 Dicember 2016).
Galante, A.M.S., Galante, O.L., Campos, L.L., 2010. Study
on application of PTFE, FEP and PFA fluoropolymers
on radiation dosimetry, Nuclear Instruments and
Methods in Physics Research 619, pp. 177-180.
Gopal, V., Goren, A., Gannot, I., 2004. Coherent hollow-
core waveguide bundles for infrared imaging, Opt.
Eng. 5 (43), pp. 1195-1199.
Infrared Detectors, 2017. Available at: http://www.
hamamatsu.com/resources/pdf/ssd/infrared_kird0001e.
pdf (Accessed: 10 May 2017).
Nishii, J., Yamashita, T., Yamagishi, T., Tanaka, C., Sone,
H., 1991. Coherent infrared fiber image bundle,
Applied physics letters 59 (21), pp. 2639-2641.
Rahman, B. M., Agrawal, A., 2013. Finite Elements
Modeling Methods for Photonics, Artech house.
Rave, E., Nagli, L., Katzir, A., 2000. Ordered bundles of
infrared-transmitting AgClBr fibers: optical
characterization of individual fibers, Optics Letters 25
(17), pp. 1237-1239.
Snopatin, G.E., Shiryaev, V.S., Plotnichenko, V.G.,
Dianov, E.M., Churbanov, M.F., 2009. High-Purity
Chalcogenide Glasses for Fiber Optics, Inorganic
Materials 45 (13), pp. 1439-1460.
Thorlabs. Stabilized Tungsten Light Sources, 2017.
Available at: https://www.thorlabs.de/drawings/b291
d2ea04f972b3-2DD86186-AF3B-B06B-E3D8818282
5A3929/SLS201L_M-Manual.pdf (Accessed: 2 June
2017).
Vitron IG3, 2014. Available at: http://www.vitron.de/
datasheets/VITRON\%20IG-3\%20Datenblatt\%20Ju
ni\%202014\%20.pdf (Accessed: 19 Dicember 2016).
Zhang, B., Zhai, C., Qi, S., Guo, W., Yang, Z., Yang, A.,
Gai, X., Yu, Y., Wang, R., Tang, D., Tao, G., Luther-
Davies, B., 2015. High-resolution chalcogenide fiber
bundles for infrared imaging, Optics Letters 40 (19),
pp. 4384-4387.
Development of Mid-IR Fiber Bundle for Thermal Imaging
265