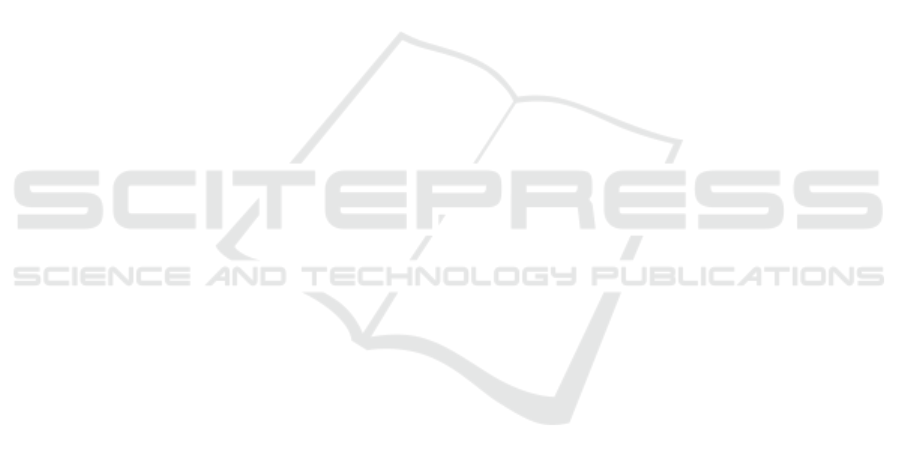
[5] Kikawada M, Ono A, Inami W and Kawata Y 2015 Surface plasmon-enhanced fluorescence
cell imaging in deep-UV region Appl. Phys. Express 8 072401
[6] Taguchi A, Hayazawa N, Furusawa K, Ishitobi H and Kawata S 2009 Deep-UV tip-enhanced
Raman scattering J. Raman Spectrosc. 40 1324-30
[7] McClain M, Schlather A, Ringe E, King N, Liu L, A Manjavacas, M Knight, I Kumar, K
Whitmire, H Everitt, P Nordlander and N Halas 2015 Aluminum nanocrystals Nano Lett.
15 2751-5
[8] Honda M, Kumamoto Y, Taguchi A, Saito Y and Kawata S 2014 Plasmon-enhanced UV
photocatalysis Appl. Phys. Lett. 104 061108
[9] Knight M, King N, Liu L, Everitt H, Nordlander P and Halas N 2014 Aluminum for
plasmonics ACS Nano 8 834-40
[10] Maidecchi G, Gonella G, Zaccaria R, Moroni R, Anghinolfi L, Giglia A, Nannarone S, Mattera
L, Dai H, Canepa M and Bisio F 2013 Deep ultraviolet plasmon resonance in Aluminum
nanoparticle arrays ACS Nano 7 5834-41
[11] Knight M, Liu L, Wang Y, Brown L, Mukherjee S, King N, Everitt H, Nordlander P and Halas
N 2012 Aluminum plasmonic nanoantennas Nano Lett. 12 6000-4
[12] Sanz J, Ortiz D, Osa R A, Saiz J, González F, Brown S, Losurdo M, Everitt H and Moreno F
2013 UV plasmonic behavior of various metal nanoparticles in the near- and far-field
regimes: geometry and substrate effects J. Phys. Chem. C 117 19606-15
[13] Tan S, Zhang L, Zhu D, Goh X, Wang Y M, Kumar K, Qiu C and Yang J 2014 Plasmonic
color palettes for photorealistic printing with Aluminum nanostructures Nano Lett. 14
4023-9
[14] Ekinci Y, Solak H and Löffler J 2008 Plasmon resonances of aluminum nanoparticles and
nanorods J. Appl. Phys. 104 083107
[15] Gérard D and Gray S 2015 Aluminium plasmonics J. Phys. D: Appl. Phys. 48 184001
[16] Kuzma A, Weis M, Flickyngerova S, Jakabovic J, Satka A, Dobrocka E, Chlpik J, Cirak J,
Donoval M, Telek P, Uherek F and Donoval D 2012 Influence of surface oxidation on
plasmon resonance in monolayer of gold and silver nanoparticles J. Appl. Phys 112 103531
[17] Gutierrez Y, Ortiz D, Sanz J, Saiz J, Gonzalez F, Everitt H and Moreno F 2016 How an oxide
shell affects the ultraviolet plasmonic behavior of Ga, Mg, and Al nanostructures Opt.
Express 24 20621-31
[18] Han M, Xu C, Zhu D, Yang L, Zhang J, Chen Y, Ding K, Song F and Wang G 2007
Controllable synthesis of two-dimensional metal nanoparticle arrays with oriented size and
number density gradients Adv. Mater. 19 2979
[19] Hu J, Chen L, Lian Z, Cao M, Li H, Sun W, Tong N and Zeng H 2012 Deep-ultraviolet blue-
light surface plasmon resonance of Al and Al-core/Al
2
O
3
shell in spherical and cylindrical
nanostructures J. Phys. Chem. C 116 15584-90
[20] Rechberger W, Hohenau A, Leitner A, Krenn J, Lamprecht B and Aussenegg F 2003 Optical
properties of two interacting gold nanoparticles Opt. Commun. 220 137-41
[21] Su K, Wei Q H, Zhang X, Mock J, Smith D and Schultz S 2003 Interparticle coupling effects
on plasmon resonances of nanogold particles Nano Lett. 3 1087-90
[22] Gong Y, Zhou Y, He L, Xie B, Song F, Han M and Wang G 2013 Systemically tuning the
surface plasmon resonance of high-density silver nanoparticle films Eur. Phys. J. D 67 87
[23] Ramaswamy A and Kaste P 2005 A 'nanovision'' of the physiochemical phenomena occurring
in nanoparticles of aluminum J. Energ. Mater. 23 1-25
[24] Levitas V, McCollum J and Pantoya M 2015 Pre-stressing micron-scale Aluminum core-shell
particles to improve reactivity Sci. Rep. 5 7879
Fabrication of Dense Aluminum Nanoparticle Arrays with Controllable Deep Ultraviolet Surface Plasmon Resonance Properties
597