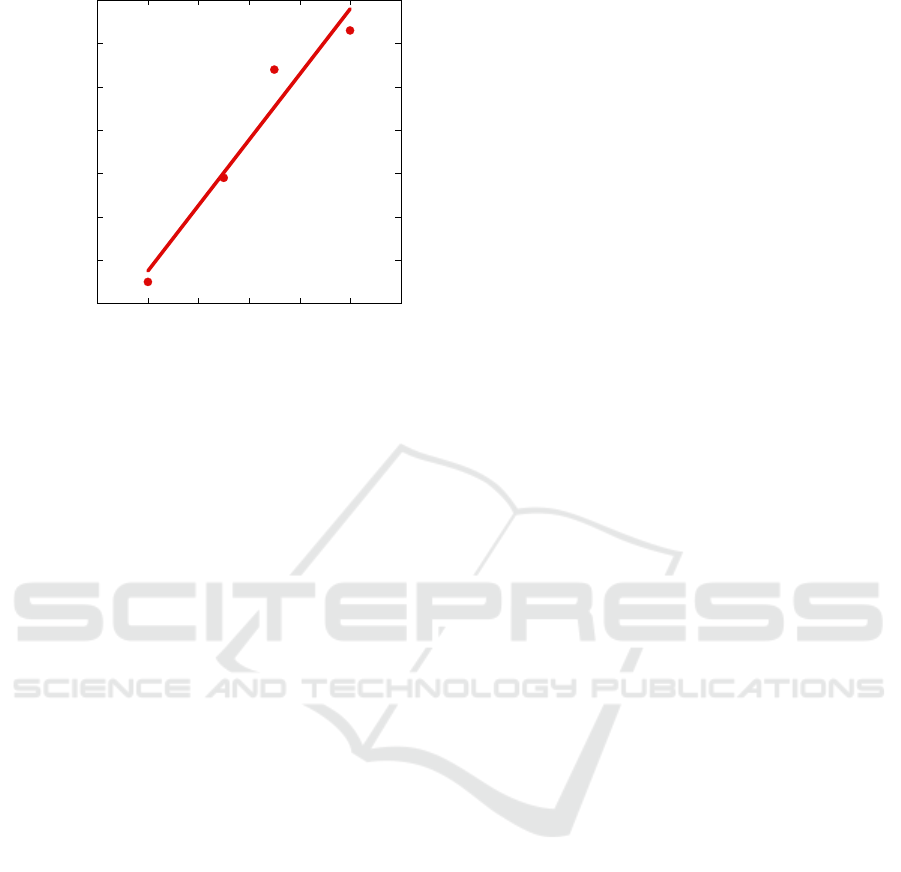
Figure 7: Device resistance variation for different NO
2
concentrations. Experimental data (points) and linear fit
(line).
We determined the sensor noise by continuously
measuring its resistance with no gas flow for 10
minutes and determining the standard deviation of the
acquired data. We employed this data to extrapolate
the theoretical detection limit of our sensor obtaining
a minimum detectable NO2 concentration of 4.9ppb.
Even if our detector shows lower performance if
compared to other PbS QD based NO
2
sensors (Song
et al., 2018), it should be noted that we propose a
device that is already integrated on a Si/SiO
2
substrate
and that, consequently, could be easily coupled with
readout electronics to produce a standalone NO2 gas
sensor with a more-than-Moore approach. Moreover,
device parameters such as QD film thickness and
metal finger spacing could be optimized to enhance
the device response. This analysis is, however, out of
the scope of this paper and will be discussed in future
publications.
5 CONCLUSIONS
In this paper we showed our results with optical and
chemical sensors based on PbS colloidal quantum
dots. We showed how the same CQD device, with
only slight modifications in the fabrication process,
can have manifold applications for different sensing
purposes. We proposed a novel fire detector with
ultra-high sensitivity for indoor applications and a
pollution gas sensor integrated on a Si/SiO
2
substrate,
defining a strategy for future sensor integration with
silicon electronics.
REFERENCES
Babrauskas, V., 1980. Estimating room flashover
potential. Fire Technology, 16(2), 94-103.
Balazs, D. M., Nugraha, M. I., Bisri, S. Z., Sytnyk, M.,
Heiss, W., and Loi, M. A., 2014. Reducing charge
trapping in PbS colloidal quantum dot solids. Applied
Physics Letters, 104(11), 112104.
Brown, P. R., Kim, D., Lunt, R. R., Zhao, N., Bawendi, M.
G., Grossman, J. C., and Bulovi, V., 2014. Energy
level modification in lead sulfide quantum dot thin
films through ligand exchange. ACS nano, 8(6), 5863-
5872.
Caruge, J. M., Halpert, J. E., Wood, V., Bulovi, V., and
Bawendi, M. G., 2008. Colloidal quantum-dot light-
emitting diodes with metal-oxide charge transport
layers. Nature photonics, 2(4), 247.
De Iacovo, A., Colace, L., Scopa, L., and Foglia, S., 2016.
Near-infrared photodetectors based on PbS colloidal
quantum dots. In Optical Sensing and Detection
IV (Vol. 9899, p. 989908). International Society for
Optics and Photonics.
De Iacovo, A., Venettacci, C., Colace, L., Scopa, L., and
Foglia, S., 2016. PbS Colloidal Quantum Dot
Photodetectors operating in the near infrared. Scientific
reports, 6, 37913.
De Iacovo, A., Venettacci, C., Colace, L., Scopa, L., and
Foglia, S., 2017. Noise performance of PbS colloidal
quantum dot photodetectors. Applied Physics
Letters, 111(21), 211104.
Kagan, C. R., Lifshitz, E., Sargent, E. H., and Talapin, D.
V. (2016). Building devices from colloidal quantum
dots. Science, 353(6302), aac5523.
Konstantatos, G., Howard, I., Fischer, A., Hoogland, S.,
Clifford, J., Klem, E., Levina, L., and Sargent, E. H.,
2006. Ultrasensitive solution-cast quantum dot
photodetectors. Nature, 442(7099), 180.
Konstantatos, G., and Sargent, E. H., 2007. PbS colloidal
quantum dot photoconductive photodetectors:
Transport, traps, and gain. Applied Physics
Letters, 91(17), 173505.
Liu, H., Li, M., Voznyy, O., Hu, L., Fu, Q., Zhou, D., Xia,
Z., Sargent, E. H., and Tang, J., 2014. Physically
flexible, rapid‐response gas sensor based on colloidal
quantum dot solids. Advanced Materials, 26(17), 2718-
2724.
Moreels, I., Lambert, K., Smeets, D., De Muynck, D.,
Nollet, T., Martins, J. C., Vanhaecke, F., Vantomme,
A., Delerue, C., Allan, G., and Hens, Z., 2009. Size-
dependent optical properties of colloidal PbS quantum
dots. ACS nano, 3(10), 3023-3030.
Pu, Y., Cai, F., Wang, D., Wang, J. X., and Chen, J. F.,
2018. Colloidal synthesis of semiconductor quantum
dots toward large-scale production: A
review. Industrial and Engineering Chemistry
Research, 57(6), 1790-1802.
Saran, R., and Curry, R. J., 2016. Lead sulphide nanocrystal
photodetector technologies. Nature Photonics, 10(2),
81.
20
30
40
50
60
70
80
90
0 10 20 30 40 50 60
R [%]
NO
2
concentration [ppmmol]
Lead Sulphide Colloidal Quantum Dots for Sensing Applications
239