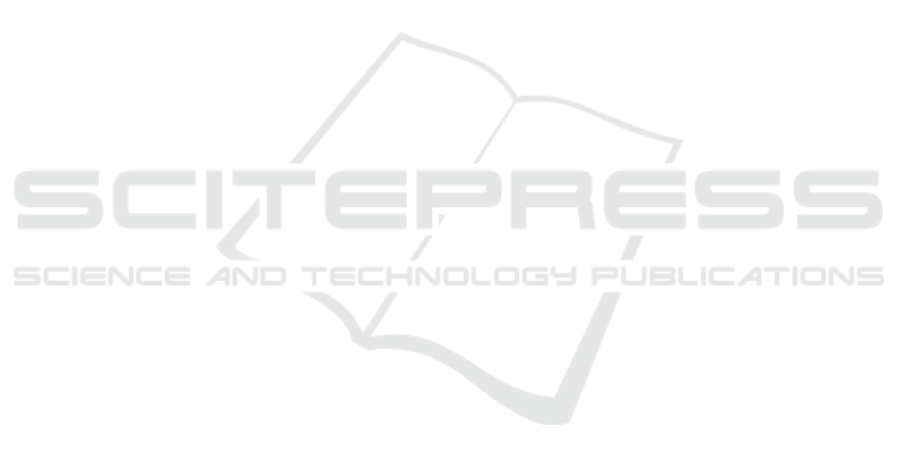
4.3 Mechanical Properties
4.3.1 Dynamic Mechanical Analysis (DMA)
The viscoelastic measurements were performed using
a DMA Q800 from TA Instruments. The
measurements were carried out at 37ºC in wet
conditions. Samples were cut in rectangular shapes
with about 14.5 x 5.3 mm (l,w) and 0.2 mm thickness
and clamped in the DMA apparatus. The sample was
deformed at constant stress-amplitude (25 µm) over 3
different frequencies (0.1, 1 and 10 Hz).
4.3.2 Tensile Tests
Mechanical properties were assessed under uniaxial
tension, using a Zwick/Roell (Leominster,
Herefordshire, UK) Z005 testing machine, loaded
with a 10 N load cell, as has been described
previously.
The samples were pre-cut into a dog-bone shape,
as per ASTM D882−2010 guidelines. Prior to testing,
all samples were incubated overnight at room
temperature in PBS and tissue paper was used to
remove excess PBS. The samples thickness was
measured using digital callipers (Scienceware, Digi-
Max, Sigma-Aldrich, Ireland). The samples were
hand-tightened between the vertical grips, which
were set at 50 mm gauge length. Scaffolds that broke
at contact points with the grips were rejected from the
analysis. The extension rate was set at 5 mm/min. The
following definitions were used to calculate
mechanical data: stress at break was defined as the
load at failure divided by the original cross-sectional
area (engineering stress), strain at break was defined
as the increase in scaffold length required to cause
failure divided by the original length, and modulus
was defined as the linear region of the stress-strain
curve using a stress at 0.02 strain divided by 0.02.
4.4 Degradation Studies
Films were incubated at 37°C in PBS for up to three
months. After predetermined periods of time samples
were removed from the solution, rinsed with distilled
water and dried at 37°C for 48 hours. Membrane mass
was weighted, and the percentage of weight loss was
calculated following equation 1:
% weight loss = (m
i
– m
f
)/m
i
X 100
Where m
i
and m
f
are the initial and final mass of
the sample, respectively.
4.5 In Vitro Assessment
4.5.1 Human Bone Marrow Stem Cells
(hBMSCs) Isolation and Culture
BMSCs were isolated according to standard
protocols. Briefly, bone marrow was flushed from
femurs and the flush-out solution was thoroughly
resuspended in complete basal medium and passed
through a 70 µm cell strainer. Cells were washed in
PBS- and were subsequently plated in complete basal
medium. After 2 days in culture non-adherent cells
were removed by several washes in PBS- and
cultured to near confluence (approx. 80%). Cells were
trypsinised, pooled, and re-plated (passage 0). Cells
were subsequently passaged at approximately 70-
80% confluency and were never allowed to reach full
confluency. Cells at passage 2 to 4 were used for all
experiments.
4.5.2 hBMSCs Seeding on Polymeric Films
Prior to cell culture studies, the films were sterilized
by ethylene oxide. hBMSCs at passages 3–4 were
harvested from culture flasks using trypLE Express
(Thermo Fisher, USA). Cells were washed with PBS
and centrifuged at 1200 rpm for 5 minutes. The cell
pellet was resuspended in α-minimal essential
medium (αMEM) supplemented with 10% fetal
bovine serum (FBS) and 2mM GlutaMAX and the
cells were seeded at different concentrations
described below onto the polymeric films, previously
placed into wells of a 24 well plate. The cells were
then cultured at 37 °C in a humidified atmosphere of
5% CO
2
. Subsequently, a drop of 100 μl of the cell
solution was seeded on top of the films and the cells
were allowed to attach at 37 °C, 5% CO2, 90%
humidity for 2,5 h prior to adding 900 μl complete
basal medium. The medium was changed every other
day.
4.5.3 Cell Adhesion
To evaluate the influence of the substrate stiffness in
cell morphology, 500 cell/cm
2
were seeded into the
polymeric films and incubated for 6 and 24 hours.
hBMSCs morphology was assessed by F-actin and
DAPI staining. Cells were fixed using a 10% formalin
solution for 30 minutes at 4°C. The samples were then
washed with PBS. For cell permeabilization it was
used a solution of 2% BSA in PBS. 4′,6-Diamidino-
2-phenylindole dihydrochloride (DAPI) and
Rhodamine B isothiocyanate were used to stain the
cell nuclei and F-actin filaments, respectively. The
samples were incubated with 1 mL of PBS containing
DCBIOSTEC 2019 - Doctoral Consortium on Biomedical Engineering Systems and Technologies
30