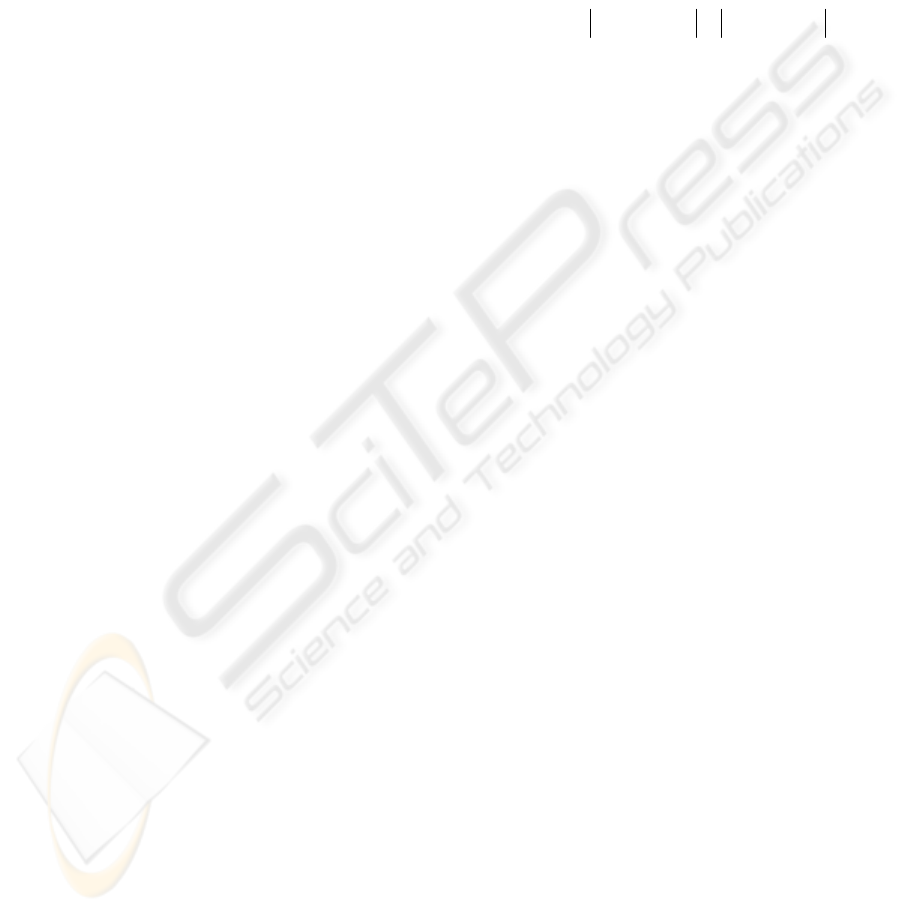
Steendijk P, Tulner SAF, Schreuder JJ, et al.
Quantification of left ventricular mechanical
dyssynchrony by conductance catheter in heart failure
patients. Am J Physiol 2004;286:H723-H730
Schumann A, Wessel N, Schirdewan A, Osterziel KJ,
Voss A. Potential of feature selection methods in heart
rate variability analysis for the classification of
different cardiovascular diseases. Stat Med. 2002 Aug
15;21(15):2225-42.
Zywietz CW, Von Einem V, Widiger B, Joseph G. ECG
analysis for sleep apnea detection. Methods Inf Med.
2004; 43(1):56-9.
Severi S, Cavalcanti S, Avanzolini G. Heart rate
variability spectral indices for haemodynamic
classification of haemodialysis patients. Physiol Meas.
1997 Nov; 18(4):339-53.
Asyali MH, Berry RB, Khoo MC, Altinok A. Determining
a continuous marker for sleep depth. Comput Biol
Med. 2007 Apr 12;
Cerutti S, Alberti M, Baselli G, Rimoldi O, Malliani A,
Merri M, Pagani M. Automatic assessment of the
interaction between respiration and heart rate
variability signal. Med Prog Technol. 1988; 14(1):7-19.
Montano N, Porta A, Malliani A. Evidence for central
organization of cardiovascular rhythms. Ann N Y
Acad Sci. 2001 Jun;940:299-306. Review.
Ropella KM, Sahakian AV, Baerman JM, Swiryn S. The
coherence spectrum. A quantitative discriminator of
fibrillatory and nonfibrillatory cardiac rhythms.
Circulation 1989;80:112-9.
Carter C, Knapp CH, Nuttall B. Estimation of the
magnitudesquared coherence function via overlapped
fast Fourier Transform processing. IEEE Trans Audio
Electroacoustics. 1973;21:337-44.
Schreuder JJ, Steendijk P, Van der Veen FH, et al. Acute
and short-term effects of partial left ventriculectomy in
dilated cardiomyopathy: assessment by pressure-
volume loops. J Am Coll Cardiol 2000;36:2104-2114.
Schreuder JJ, van der Veen FH, van der Velde ET, et al.
Left ventricular pressure-volume relationships before
and after cardiomyoplasty in patients with heart
failure. Circulation. 1997;96:2978-2986.
Leclercq C, Kass DA. Retiming the failing heart:
principles and current clinical status of cardiac
resynchronization. J Am Coll Cardiol 39: 194–201, 2002.
APPENDIX
Mechanical dyssynchrony. At each time point, a
segmental signal is defined as dyssynchronous if its
change (i.e., dSV/dt) is opposite to the simultaneous
change in the total LV volume (dTV/dt). Segmental
dyssynchrony is quantified by calculating the
percentage of time within the cardiac cycle that a
segment is dyssynchronous. Overall LV
dyssynchrony (DYS) is calculated as the mean of the
segmental dyssynchronies. DYS may be calculated
within each specified time interval, i.e. during
systole and diastole, with systole defined as the
period between the moments of dP/dtmax and
dP/dtmin.
Internal flow. Nonuniform contraction and filling
is associated with ineffective shifting of blood
volume within the LV. This internal flow (IF) is
quantified by calculating the sum of the absolute
volume changes of all segments and subtracting the
absolute total volume change:
2//)(/)()(
−= dttdTVdttdSVitIF
Note that dTV(t)/dt represents the effective flow into
or out of the LV. Thus IF measures the segment-to-
segment blood volume shifts, which do not result in
effective filling or ejection. Division by two takes
into account that any non-effective segmental
volume change is balanced by an equal but opposite
volume change in the remaining segments. IF
fraction (IFF) is calculated by integrating IF(t) over
the full cardiac cycle and dividing by the integrated
absolute effective flow.
Mechanical dispersion. In the HF patients, a
substantial dispersion is present in the onset of
contraction between the segments. This dispersion is
assessed by segmental lag times which are
determined by calculating the cross correlations
between TV(t) and SV(t) for all systolic time points
(i.e., between dP/dtmax and dP/dtmin). For each
segment the lag which produces the highest linear
correlation is determined. Mechanical dispersion
(DISP) is defined as 2 standard deviation of the
segmental lag times. Recently, new parameters have
been introduced to quantify LV dyssynchrony with
echocardiographic techniques. These indices can be
directly applied to conductance method.
Cycle Efficiency. Calculated as previously described
by the formula: CE=SW/[ LVP* LV volume], with
SW = stroke work, LVP = end-systolic – end-
diastolic LV pressure. This index quantifies
distortions in the shape of the pressure-volume
diagram. The calculation assumes that the optimal
contraction would have CE value near 1.0,
corresponding to a rectangular pressure volume
diagram. Decreases in cycle efficiency may be
caused by multiple factors including isovolumic
volume shifts as well as changes in afterload and
ventricular stiffness. Similarly, regional cycle
efficiency can be calculated from the most basal to
the most apical segmental volume signal plotted
against LV pressure. Differences in regional cycle
efficiency during isovolumic filling or emptying
may indicate inefficient patterns contraction or
relaxation due to dyssynchrony.
SPECTRAL AND CROSS-SPECTRAL ANALYSIS OF CONDUCTANCE CATHETER SIGNALS - New Indexes for
Quantification of Mechanical Dyssinchrony
443