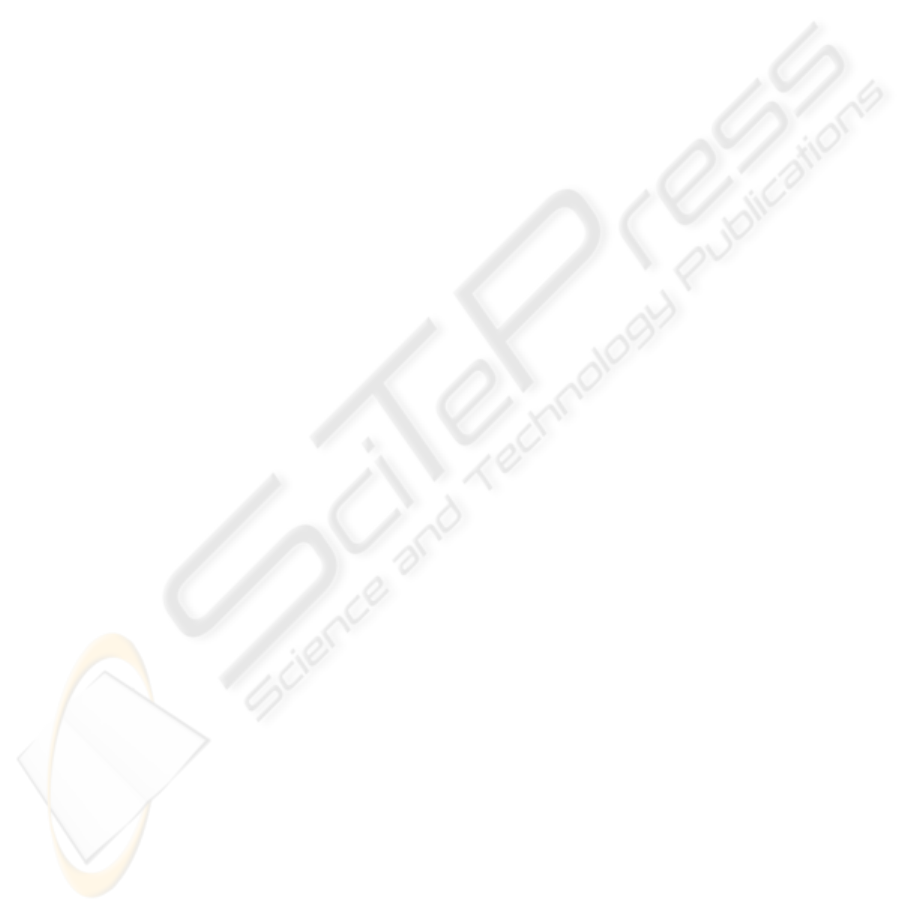
be replaced by a final FACS for proper composition
of the tethered assemblies. By introducing an inter-
mediate separation process, a feedback of single
vesicles and incomplete assemblies into the self-
assembly process may be realized before they be-
come encapsulated (Figure 3 E). This may increase
encapsulation efficiency and therefore may econo-
mize the production of custom-tailored vesicular
drug delivery systems.
Encapsulation provides an extended circulation
time resulting in accumulation at tumors or inflam-
mation sites due the EPR effect, without the need of
specific targeting. On the other hand, multiple com-
partments offer segregation of multicomponent
pharmaceuticals that might be released only when
and where they are needed. Permeability control
might be realized either by exploitation of stimuli
inherent to target site (pH, redox potential, tempera-
ture) or externally induced (temperature, magnetic
field, ultrasound). For a recent review on stimuli-
sensitive pharmaceutical nanocarriers see Torchilin
(2009).
5 CONCLUSIONS
Encapsulated multicompartment systems may pro-
vide stable vehicles for a multicomponent or multi-
functional personalized drug delivery. In this work,
we established a novel encapsulation technique and
provide evidence for the first stable DNA-mediated
linkage of more than two vesicle populations. We
discussed how these techniques may personalize the
individual healthcare by providing custom-tailored
vesicular drug delivery systems.
ACKNOWLEDGEMENTS
Maik Hadorn was supported by the Swiss National
Foundation Project 200020-118127 Embryogenic
Evolution: From Simulations to Robotic Applica-
tions. Peter Eggenberger Hotz was partly supported
by the European Union integrated project PACE
(EU-IST-FP6-FET-002035). We thank Eva Bönzli
for careful reading of the manuscript.
REFERENCES
Allen, T. M. and Cullis, P. R. (2004). Drug delivery sys-
tems: Entering the mainstream. Science, 303(5665),
1818-1822.
Bakker-Woudenberg, I., Schiffelers, R. M., Storm, G.,
Becker, M. J. and Guo, L. (2005). Long-circulating
sterically stabilized liposomes in the treatment of in-
fections, Liposomes, Pt E (391: 228-260). San Diego:
Elsevier Academic Press Inc.
Beales, P. A. and Vanderlick, T. K. (2007). Specific bind-
ing of different vesicle populations by the hybridiza-
tion of membrane-anchored DNA. Journal of Physical
Chemistry A, 111(49), 12372-12380.
Benkoski, J. J. and Hook, F. (2005). Lateral mobility of
tethered vesicle - DNA assemblies. Journal of Physi-
cal Chemistry B, 109(19), 9773-9779.
Berti, D., Baglioni, P., Bonaccio, S., Barsacchi-Bo, G. and
Luisi, P. L. (1998). Base complementarity and nucleo-
side recognition in phosphatidylnucleoside vesicles.
Journal of Physical Chemistry B, 102(1), 303-308.
Bolinger, P. Y., Stamou, D. and Vogel, H. (2008). An
integrated self-assembled nanofluidic system for con-
trolled biological chemistries. Angewandte Chemie-
International Edition, 47(30), 5544-5549.
Bonacucina, G., Cespi, M., Misici-Falzi, M. and Palmieri,
G. F. (2009). Colloidal Soft Matter as Drug Delivery
System. Journal of Pharmaceutical Sciences, 98(1),1-42.
Boyer, C. and Zasadzinski, J. A. (2007). Multiple lipid
compartments slow vesicle contents release in lipases
and serum. Acs Nano, 1(3), 176-182.
Burridge, K. A., Figa, M. A. and Wong, J. Y. (2004).
Patterning adjacent supported lipid bilayers of desired
composition to investigate receptor-ligand binding un-
der shear flow. Langmuir, 20(23), 10252-10259.
Chan, Y. H. M., van Lengerich, B. and Boxer, S. G.
(2009). Effects of linker sequences on vesicle fusion
mediated by lipid-anchored DNA oligonucleotides.
Proceedings of the National Academy of Sciences of
the United States of America, 106(4), 979-984.
Chiruvolu, S., Walker, S., Israelachvili, J., Schmitt, F. J.,
Leckband, D. and Zasadzinski, J. A. (1994). Higher-
order self-assembly of vesicles by site-specific bind-
ing. Science, 264(5166), 1753-1756.
Constable, E. C., Meier, W., Nardin, C. and Mundwiler, S.
(1999). Reversible metal-directed assembly of clusters
of vesicles. Chemical Communications,(16), 1483-
1484.
Eckstein, F. (2007). The versatility of oligonucleotides as
potential therapeutics. Expert Opinion on Biological
Therapy, 7(7), 1021-1034.
Green, N. M. (1990). Avidin and streptavidin. Methods in
Enzymology, 184, 51-67.
Hotani, H., Nomura, F. and Suzuki, Y. (1999). Giant
liposomes: from membrane dynamics to cell morpho-
genesis. Current Opinion in Colloid & Interface Sci-
ence, 4(5), 358-368.
Jesorka, A. and Orwar, O. (2008). Liposomes: Technolo-
gies and Analytical Applications. Annual Review of
Analytical Chemistry, 1, 801-832.
Kisak, E., Coldren, B., Evans, C., Boyer, C. and Zasadzin-
ski, J. (2004). The vesosome-A multicompartment
drug delivery vehicle. Current medicinal chemistry,
11(2), 199-220.
TOWARDS PERSONALIZED DRUG DELIVERY - Preparation of an Encapsulated Multicompartment System
11