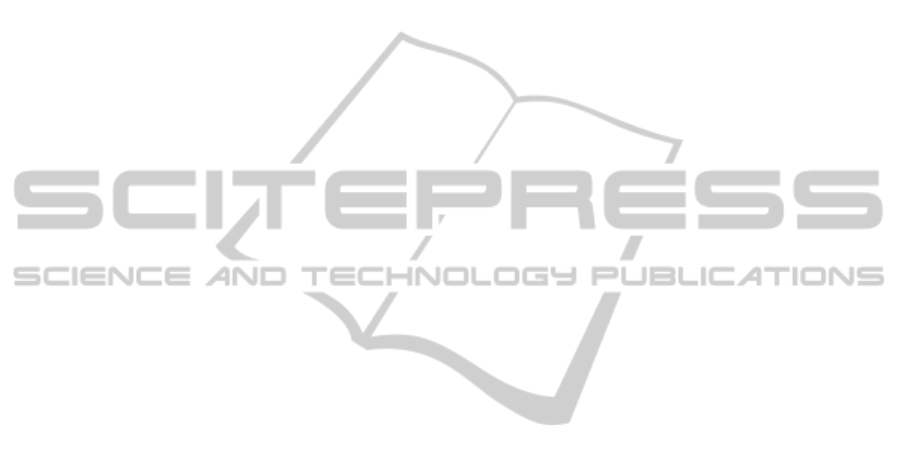
REFERENCES
Agah, A., Hassibi, A., Plummer, J., Griffin, P., 2005.
Design requirements for integrated biosensor arrays.
In Proc. SPIE 5699, Imaging, Manipulation, and
Analysis of Biomolecules and Cells: Fundamentals
and Applications III.
Balasubramanian, V., Ruedi, P.-F., Temiz, Y., Ferretti,
A., Guiducci, C., Enz, C.C., 2013. A 0.18
mbiosensor front-end based on 1/f noise, distortion
cancelation and chopper stabilization techniques. In
Biomedical Circuits and Systems, IEEE
Transactions on, vol.PP, no.99, pp.1,14.
Ferrari, G., Gozzini, F., Molari, A., Sampietro, M., 2009.
Transimpedance Amplifier for High Sensitivity
Current Measurements on Nanodevices. In Solid-
State Circuits, IEEE Journal of, vol.44, no.5,
pp.1609,1616.
Figueiredo, M., Santin, E., Goes,J., Santos-Tavares, R.,
Evans, G., 2010. Two-stage fully-differential
inverter-based self-biased CMOS amplifier with
high efficiency. In Circuits and Systems (ISCAS),
Proceedings of 2010 IEEE International Symposium
on , vol., no., pp.2828-2831, May 30 2010-June 2
2010.
Hassibi, A., Vikalo, H., Hajimiri, A., 2007. On Noise
Processes and Limits of Performance in Biosensors.
In Journal of Applied Physics, vol. 102, issue 1,
2007.
Henze, D. A., Borhegyi, Z., Csicvari, J., Mamiya, A.,
Harris, K. D., Buzsáki, G., 2000. Intracellular
Features Predicted by Extracellular Recordings in
the Hippocampus in Vivo. In Journal of
Neurophysiology, Jul. 2000, pp. 390-400.
Kinget, Peter R., 2005. Device Mismatch and Tradeoffs
in the Design of Analog Circuits. In IEEE JSSC,
Vol. 40, No. 6, June 2005.
Liao, Y., et al., 2012. A 3-µW CMOS Glucose Sensor
for Wireless Contact-Lens Tear Glucose Monitoring.
In IEEE JSSC, Vol. 47, No. 1, Jan. 2012.
Niwa, O., et al. 1998. Small-Volume On-Line Sensor for
Continuous Measurement of -Aminobutyric Acid.
In Analytical Chemistry, 1998, 70, 89-93.
Pettine, W., Jibson, M., Chen, T. Tobet, S., Henry, C.,
2012. Charaterization of novel microelectrode
geometries for detection of neurotransmitters. In
IEEE Sensors Journal, Vol.12, No. 5, May, 2012.
Pihel, K., et al., 1996. Overoxidized Polypyrrole-Coated
Carbon Fiber Microelectrodes for Dopamine
Measurements with Fast-Scan Cyclic Voltammetry.
In Anal. Chem. 1996, 68, pp. 2084-2089.
Qi, P., et al., 2003. Toward Large Arrays of Multiplex
Functionalized Carbon Nanotube Sensors for Highly
Sensitive and Selective Molecular Detection. In
Nano Lett, 2003, No. 2. 2003.
Razavi, B., 2000. A 622 Mb/s 4.5 pA//spl radic/Hz
CMOS transimpedance amplifier [for optical
receiver front-end]. In Solid-State Circuits
Conference, 2000. Digest of Technical Papers.
ISSCC. 2000 IEEE International , vol., no.,
pp.162,163, 9-9 Feb. 2000.
Rosenstein, J., Sorgenfrei, S., Shepard, K.L., 2011.
Noise and bandwidth performance of single-
molecule biosensors. In Custom Integrated Circuits
Confer
ence (CICC), 2011 IEEE , vol., no., pp.1,7,
19-21 Sept. 2011.
Salvia, J., Lajevardi, P., Hekmat, M., Murmann, B.,
2009. A 56M CMOS TIA for MEMS applications.
In Custom Integrated Circuits Conference, 2009.
CICC '09. IEEE , vol., no., pp.199,202, 13-16 Sept.
2009.
Sharma, A., Zaman, M.F., Ayazi, F., 2007. A 104-dB
Dynamic Range Transimpedance-Based CMOS
ASIC for Tuning Fork Microgyroscopes. In Solid-
State Circuits, IEEE Journal of , vol.42, no.8,
pp.1790,1802, Aug. 2007.
Starkey, S., et al., 2001. A rapid and transient synthesis
of nitric oxide (NO) by a constitutively expressed
type II NO synthase in the guinea-pig
suprachiasmatic nucleus. In British Journal of
Pharmacology (2001) 134, 1084-1092.
Tang,Y., Zhang, Y., Fedder, G.K., Carley, L.R., 2012.
An ultra-low noise Switched Capacitor
Transimpedance Amplifier for parallel Scanning
Tunneling Microscopy. In Sensors, 2012 IEEE , vol.,
no., pp.1,4, 28-31 Oct. 2012.
Toumazou, C., et al., 2002 Tradeoffs in Analog Circuit
Design. Springer, 2002.
Villagrasa, J.P., Colomer-Farrarons, J., Miribel P.L.,
2013. Bioelectronics for Amperometric Biosensors,
State of the Art in Biosensors - General Aspects, Dr.
Toonika Rinken (Ed.), ISBN: 978-953-51-1004-0,
InTech.
Wilson, W., Chen, T., Selby, R., 2013. A current-starved
inverter-based differential amplifier design for ultra-
low power applications. In Circuits and Systems
(LASCAS), 2013 IEEE Fourth Latin American
Symposium on, vol., no., pp.1,4, Feb. 27-March 1,
2013.
Wightman, R Mark, 2006. Probing Cellular Chemistry
in Biological Systems with Microelectrodes. In
Science, 17 March 2006, Vol. 311.
Xu, C., Lemon, W., and Lui, C., 2002. Design and
Fabrication of a High Density Metal Microelectrode
Array for Neural Recording. In Sensors and
Actuators, Vol. A 96, Issue. 1. pp. 78-85, Jan. 2002.
Yao, J., Gillis, K., 2012. Quantification of Noise Sources
for Amperometric Measurement of Quantal
Exocytosis Using Microelectrodes. In Analyst, vol.
137, issue 11, pp.2674-2681. ROYAL SOCIETY OF
CHEMISTRY.
Zand, B., Phang, K., Johns, D.A., 2001. A
transimpedance amplifier with DC-coupled
differential photodiode current sensing for wireless
optical communications. In Custom Integrated
Circuits, 2001, IEEE Conference on. , vol., no.,
pp.455,458, 2001.
Designofa50MOTransimpedanceAmplifierwith0.98fa/vHzInputInferredNoiseina0.18µMCMOSTechnology
119