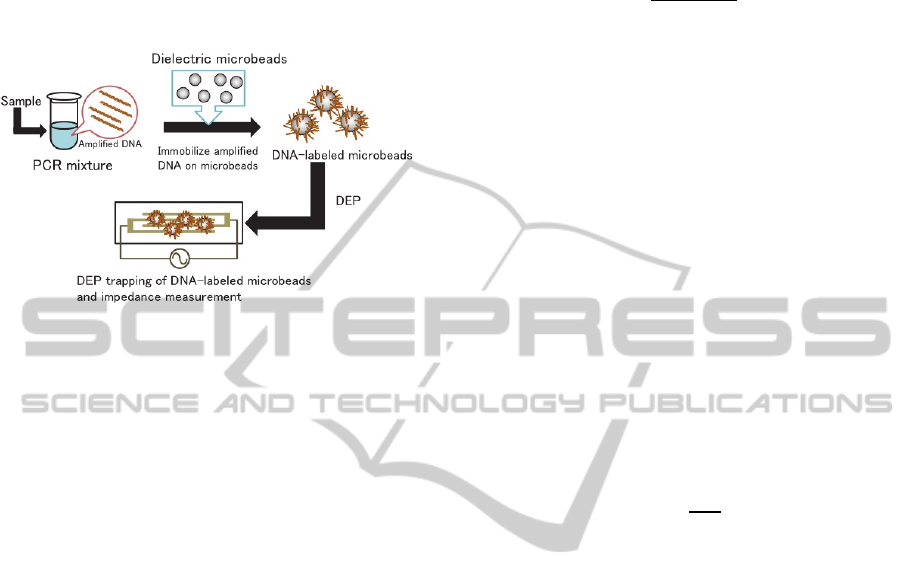
al., 2014). Hence, this method would provide rapid
detection of DNAs amplified by PCR, which may be
applicable to rapid, quantitative, and automated
diagnosis of bacterial and viral infections. However,
this method still required separation and selective
detection of PCR amplified DNA of different length.
Figure 1: Schematic illustration of the microbeads-based
detection of amplicons. After PCR amplification,
amplicons (amplified DNA) are chemically immobilized
on the microbeads. The DNA-labeled microbeads behaves
positive DEP, whereas the pristine microbeads behaves
negative DEP. The DEP-trapped microbeads can be
detected by DEPIM
This study aim to reveal how the length of
labelling DNA affects DEP characteristics of DNA
labeled microbeads. Alteration of DNA-labeled
microbeads DEP characteristics, which is affected
by the length of labeled DNA, can lead to rapid
separation and selective detection of PCR amplified
DNA of different length by impedance
measurement. Crossover frequency of the DEP for
microbeads labeled with DNA of different length
were measured at different suspending medium
conductivities. The alteration of DEP charateristics
was analysed using theoritical fitting of measured
data.
2 THEORY
DEP is the electrokinetic motion of dielectrically
polarized materials in non-uniform electric fields,
and it is currently an active area of research for
manipulation of biological particles and
nanomaterials, including bacterial cells and DNA
molecules (Pethig, 2010, Hughes, 2000, Ausbury et
al., 2002,). The DEP force acting on a spherical
dielectric particle of radius r suspended in a medium
of absolute permittivity ε
m
is given by as follows
2
Re
(1)
where E is the magnitude of the applied field.
Re[K(ω)] is the real component of the Clausius–
Mossotti (CM) factor, given by
∗
∗
∗
2
∗
(2)
where ε*
p
and ε*
m
are the complex permittivities of
the particle and the surrounding medium,
respectively. For a real dielectric, the complex
permittivity is defined as ε
*
ε‐j
σω
⁄
, where ε is
the permittivity, σ is the conductivity of the
dielectric, and ω is the angular frequency of the
applied electric field. When Re[K(ω)] has a positive
value, the particle is propelled toward the high field
region (positive DEP, p-DEP). With a negative value
of Re[K(ω)], the particle is repelled from the high
field region (negative DEP, n-DEP). The crossover
frequency f
x
is defined as the value of the applied
frequency which results in the cessation of particle
motion. Therefore, measurement of the crossover
frequency can used to characterize the dielectric
properties of single particle.
The conductivity of a solid dielectric particle, σ
p
,
can be expressed by the following equation
(Ermolina and Morgan, 2005).
2
(3)
where σ
b
and K
s
are the bulk conductivity and the
surface conductance of the particle. Equations 1–3
imply that the dielectric properties and the resultant
DEP force acting on a smaller particle should be
more dependent on the surface conductance K
s
.
Hughes et al. reported that antibody (protein)
coating of submicrometer latex spheres altered the
surface conductance and DEP spectrum of the
particles, enabling the separation of unlabeled and
protein-labeled particles (Hughes and Morgan,
1999). Zhou et al. found that the dielectric properties
of microbeads were modified by coating with
bacterial biofilms, resulting in an altered
electrorotation spectrum (Zhou et al., 1995).
3 EXPERIMENTS
We used pUC 19 DNA as template for PCR. The 5’
end of forward primers, which were designed for
amplifications of 204 bp DNA, 391 bp DNA and
796 bp DNA from pUC 19, were tagged with biotin.
As the results of PCR, 204 bp DNA, 391 bp DNA
and 796 bp DNA were amplified and these
amplicons were confirmed by standard agarose gel
electrophoresis.
BIODEVICES2015-InternationalConferenceonBiomedicalElectronicsandDevices
186