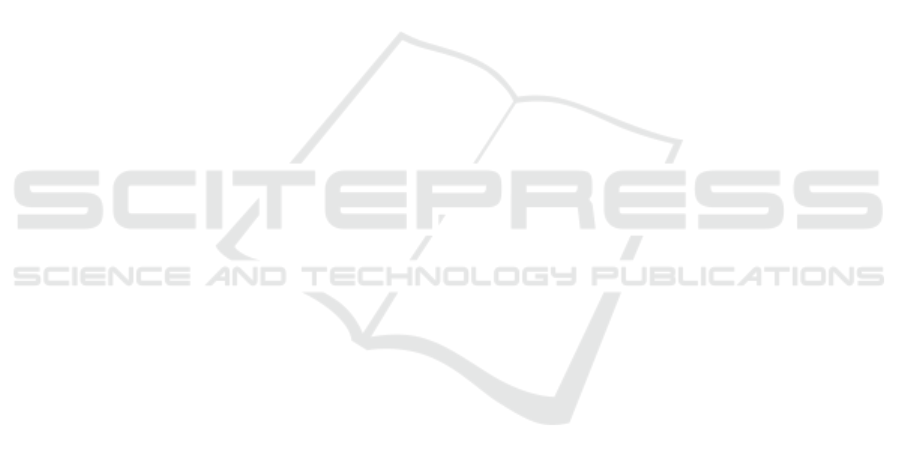
response must be normalized, and several
classification methods can be explored.
Furthermore, small, lightweight and low-power
pumps can be used in future versions of the device, so
it can achieve portability without the need of an
electric generator.
In what concerns to the electrical part, the
conductivity meter architecture should be re-designed
to be simpler and easily scalable. For instance, the
possibility of generating an input digital wave should
be explored. In addition, the electrodes finishing
surface should be more corrosion-resistant (the use of
nickel and gold, or platinum might be a solution). The
duration of the experiments related to the electrical
signals should be longer, allowing the study of the
response at different measurement intervals, to
improve quantification and interpretation of results.
The studies performed so far intend to
characterize the device, identify its limitations, and
optimize the technology. That is why, the tests were
performed successively in cycles during several
minutes. Nevertheless, our vision is to achieve a
device that gives a response in the range of seconds.
The final goal is to accomplish a portable and
user-friendly device. Accuracy, stability, and
scalability are imperative to get to an E-nose, that can
be explored towards several applications in
environmental protection, security, product quality,
or medical research.
ACKNOWLEDGMENTS
This work was supported by the European Research
Council (SCENT-ERC-2014-STG-639123) and
UCIBIO, financed by FCT/MEC (UID/Multi/
04378/2013) and co-financed by the ERDF under the
PT2020 Partnership Agreement (POCI-01-0145-
FEDER-007728). The authors thank FCT/MEC for
the research fellowship PD/BD/105752/2014 for A.P.
The authors also acknowledge funding from CNPq,
Brazil (400740/2014-1).
REFERENCES
Barsan, N., Koziej, D. and Weimar, U. (2007) ‘Metal oxide-
based gas sensor research: How to?’, Sensors and
Actuators B: Chemical, 121(1), pp. 18–35.
Boden, N. et al. (1999) ‘Device applications of charge
transport in discotic liquid crystals’, Journal of
Materials Chemistry, 9, pp. 2081–2086.
Feng, L. et al. (2010) ‘A colorimetric sensor array for
identification of toxic gases below permissible
exposure limits’, Chemical Communications, (12), pp.
2037–2039.
van Geffen, W. H., Bruins, M. and Kerstjens, H. A. M.
(2016) ‘Diagnosing viral and bacterial respiratory
infections in acute COPD exacerbations by an
electronic nose: a pilot study.’, Journal of breath
research. IOP Publishing, 10(3), p. 36001.
Gutierrez, J. and Horrillo, M. C. (2014) Advances in
artificial olfaction: Sensors and applications, Talanta.
doi: 10.1016/j.talanta.2014.02.016.
Gutmacher, D. et al. (2011) ‘Comparison of gas sensor
technologies for fire gas detection’, Procedia
Engineering. Elsevier B.V., 25, pp. 1121–1124.
van Hooren, M. R. A. et al. (2016) ‘Differentiating head
and neck carcinoma from lung carcinoma with an
electronic nose: a proof of concept study’, European
Archives of Oto-Rhino-Laryngology, 273(11), pp.
3897–3903.
Hussain, A. et al. (2017) ‘Tunable Gas Sensing Gels by
Cooperative Assembly’, Advanced Functional Mate-
rials, 27(27), pp. 1–14. Available at: http://dx.doi.org/
10.1002/adfm.201700803.
Jing Kong, author et al. (2000) ‘Nanotube Molecular Wires
as Chemical Sensors’, 287(5453 OP-Science.
287(5453):622-625), p. 622.
Li, S. (2009) Overview of Odor Detection Instrumentation
and the Potential for Human Odor Detection in Air
Matrices. MITRE Nano.
Llobet, E. et al. (1999) ‘Fuzzy ARTMAP based electronic
nose data analysis’, Sensors & Actuators: B. Chemical,
61(1 OP), pp. 183–190.
Mirzaei, A., Leonardi, S. G. and Neri, G. (2016) ‘Detection
of hazardous volatile organic compounds (VOCs) by
metal oxide nanostructures-based gas sensors: A
review’, Ceramics International. Elsevier, 42(14), pp.
15119–15141. doi: 10.1016/j.ceramint.2016.06.145.
Persaud, K. and Dodd, G. (1982) ‘Analysis of discrimina-
tion mechanisms in the mammalian olfactory system
using a model nose.’, Nature, 299(5881), pp. 352–5.
da Rocha, R. T., Gutz, I. G. R. and do Lago, C. L. (1997)
‘A Low-Cost and High-Performance Conductivity
Meter’, Journal of Chemical Education. American
Chemical Society, 74(5), p. 572. Available at:
http://dx.doi.org/10.1021/ed074p572.
Wilkens, W. F. and Hartman, J. D. (1964) ‘An electronic
analog for the olfactory processes’, Annals New York
Academy of Sciences, (512), pp. 608–612.
Zohora, S. E., Srivastava, A. K. and Dey, N. (2016) ‘Gas
Sensing Techniques in Electronic Nose and its
Applications : A Review’, EEECOS, pp. 178–183.
Design and Evolution of an Opto-electronic Device for VOCs Detection
55