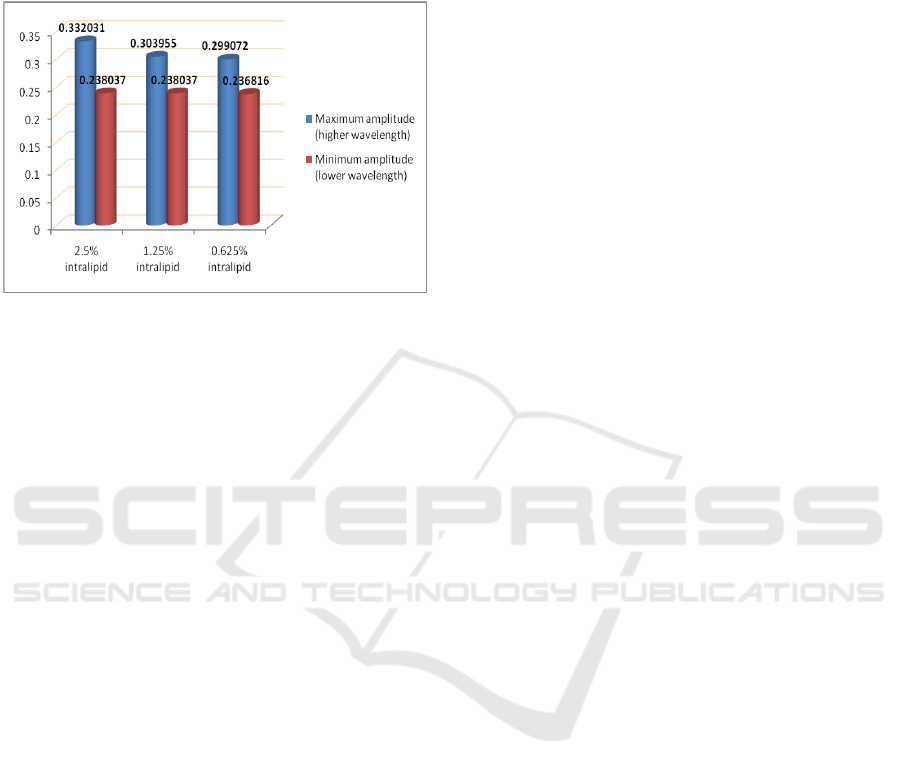
backscattering is linked to the structural and
functional parameters of tissues. These analyses are
required for characterization of optical phantoms in
medical applications.
Figure 10: Amplitude profile for the three phantoms.
4 CONCLUSIONS
The work presented here describes the preparation of
solid static phantoms of agarose and intralipid. They
are tested using single-channel time domain NIRS
system. The phantom design presented is easy to
prepare and can be used to get inhomogeneous
samples with preferred optical properties. Using
similar procedures, it is possible to get multi-layered
structure with varying optical properties so as to
mimic real brain tissues. The NIRS system design is
based on a dual‐core ARM Cortex‐A9 processor
(myRIO Student Embedded Device) and have high
speed NIRS data acquisition rate. For this study, we
attempted to see the affect of scattering agent in the
medium on photo detector output. Similar attempts
can be made on sophisticated designed phantoms
that replicate the real brain. Such experiments
provide an insight in analysis of in vitro complex
structures.
ACKNOWLEDGEMENTS
This work was supported by DST-DAAD project
(POCT-NIRS), Indian Institute of Technology
Mandi, MeitY, Govt of India and University
Medical Center Freiburg. The authors would like to
thank Prof. Dr. Ulrich G. Hofmann, Rand K.
Almajidy for their significant suggestions and help
regarding this work.
REFERENCES
Arora, Y., Sharma, G., Jindal, U., Sood, M., Dutta, A.,
Das, A. and Chowdhury, S.R., 2016, October. A low
cost non invasive hardware for anodal transcranial
direct current stimulation to screen stroke patients
using continuous wave functional near infrared
spectroscopy. In international journal of stroke (Vol.
11, no. Supp 3, pp. 275-275). 1 Olivers yard, 55 City
road, London EC1Y 1sp, England: sage publications
ltd.
Biswal, N.C., Xu, Y. and Zhu, Q., 2011. Imaging tumor
oxyhemoglobin and deoxyhemoglobin concentrations
with ultrasound-guided diffuse optical
tomography. Technology in cancer research &
treatment, 10(5), pp.417-429.
Cubeddu, R., Pifferi, A., Taroni, P., Torricelli, A. and
Valentini, G., 1997. A solid tissue phantom for photon
migration studies. Physics in medicine and
biology, 42(10), p.1971.
Dutta, A., Jacob, A., Chowdhury, S.R., Das, A. and
Nitsche, M.A., 2015. EEG-NIRS based assessment of
neurovascular coupling during anodal transcranial
direct current stimulation-a stroke case series. Journal
of medical systems, 39(4), p.36.
Hielscher, A.H., Liu, H., Chance, B., Tittel, F.K. and
Jacques, S.L., 1996. Time-resolved photon emission
from layered turbid media. Applied optics, 35(4),
pp.719-728.
Jindal, U., Sood, M., Dutta, A. and Chowdhury, S.R.,
2015. Development of point of care testing device for
neurovascular coupling from simultaneous recording
of EEG and NIRS during anodal transcranial direct
current stimulation. IEEE journal of translational
engineering in health and medicine, 3, pp.1-12.
Jobsis, F.F., 1977. Noninvasive, infrared monitoring of
cerebral and myocardial oxygen sufficiency and
circulatory parameters. Science, 198(4323), pp.1264-
1267.
Lin, Y., Lech, G., Nioka, S., Intes, X. and Chance, B.,
2002. Noninvasive, low-noise, fast imaging of blood
volume and deoxygenation changes in muscles using
light-emitting diode continuous-wave imager. Review
of Scientific Instruments, 73(8), pp.3065-3074.
Lindquist, C., Pifferi, A., Berg, R., Andersson‐Engels, S.
and Svanberg, S., 1996. Reconstruction of diffuse
photon‐density wave interference in turbid media from
time‐resolved transmittance measurements. Applied
physics letters, 69(12), pp.1674-1676.
Madsen, S.J., Wilson, B.C., Patterson, M.S., Park, Y.D.,
Jacques, S.L. and Hefetz, Y., 1992. Experimental tests
of a simple diffusion model for the estimation of
scattering and absorption coefficients of turbid media
from time-resolved diffuse reflectance
measurements. Applied optics, 31(18), pp.3509-3517.
Markus, H. and Cullinane, M., 2001. Severely impaired
cerebrovascular reactivity predicts stroke and TIA risk
in patients with carotid artery stenosis and
occlusion. Brain, 124(3), pp.457-467.
BIODEVICES 2018 - 11th International Conference on Biomedical Electronics and Devices
162