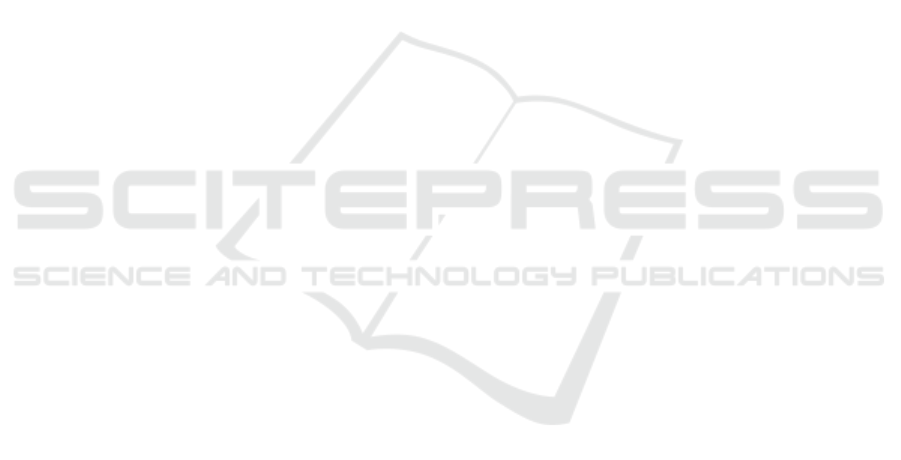
ACKNOWLEDGEMENTS
The work of this paper was funded by the Euro-
pean Regional Development Fund under the operation
number ‘ZS /2016/04/78123’ as part of the initiative
“Sachsen-Anhalt WISSENSCHAFT Schwerpunkte”.
The authors have no conflict of interest.
REFERENCES
Ab
¨
acherli, R., Pasquier, C., Odille, F., Kraemer, M.,
Schmid, J., and Felblinger, J. (2005). Suppression of
MR gradient artefacts on electrophysiological signals
based on an adaptive real-time filter with LMS coeffi-
cient updates. MAGMA, 18(1):41–50.
Abi-Abdallah, D., Chauvet, E., Bouchet-Fakri, L., Batail-
lard, A., Briguet, A., Fokapu, O., et al. (2006). Ref-
erence signal extraction from corrupted ECG using
wavelet decomposition for MRI sequence triggering:
application to small animals. Biomed Eng Online,
5(1):1–12.
Ashikaga, H., Sasano, T., Dong, J., Zviman, M. M., Evers,
R., Hopenfeld, B., Castro, V., Helm, R. H., Dickfeld,
T., Nazarian, S., et al. (2007). Magnetic resonance–
based anatomical analysis of scar-related ventricular
tachycardia: implications for catheter ablation. Circu-
lation research, 101(9):939–947.
Barkhausen, J., Kahn, T., Krombach, G. A., Kuhl, C. K.,
Lotz, J., Maintz, D., Ricke, J., Schoenberg, S. O.,
Vogl, T. J., Wacker, F. K., et al. (2017). White Pa-
per: Interventional MRI: Current Status and Potential
for Development Considering Economic Perspectives,
Part 1: General Application. In R
¨
oFo-Fortschritte auf
dem Gebiet der R
¨
ontgenstrahlen und der bildgeben-
den Verfahren, volume 189, pages 611–623.
Bhatt, B. and Reddy, M. (2009). ICA Based Flow Artifact
Removal from ECG during MRI. In Proc Int Conf
ACT 09, pages 241–243.
Chubb, H., Williams, S. E., Whitaker, J., Harrison, J. L.,
Razavi, R., and O’Neill, M. (2017). Cardiac electro-
physiology under mri guidance: an emerging technol-
ogy. Arrhythm Electrophysiol Rev, 6(2):85–93.
Dukkipati, S., Mallozzi, R., Schmidt, E., Holmvang, G.,
Avila, A., Guhde, R., Darrow, R., Slavin, G., Fung,
M., Malchano, Z., et al. (2008). Electroanatomic
Mapping of the Left Ventricle in a Porcine Model
of Chronic Myocardial Infarction With Magnetic
Resonance-Based Catheter Tracking. Circulation,
118(8):853–862.
Dzeshka, M. S., Lip, G. Y., Snezhitskiy, V., and Shantsila,
E. (2015). Cardiac fibrosis in patients with atrial fib-
rillation: mechanisms and clinical implications. J Am
Coll Cardiol, 66(8):943–959.
Elbes, D., Magat, J., Govari, A., Ephrath, Y., Vieillot, D.,
Beeckler, C., Weerasooriya, R., Jais, P., and Quesson,
B. (2017). Magnetic resonance imaging-compatible
circular mapping catheter: an in vivo feasibility and
safety study. EP Europace, 19(3):458–464.
Felblinger, J., Slotboom, J., Kreis, R., Jung, B., Boesch, C.,
et al. (1999). Restoration of Electrophysiological Sig-
nals Distorted by Inductive Effects of Magnetic Field
Gradients During MR Sequences. Magnet Reson Med,
41(4):715–721.
Fischbach, F., Lohfink, K., Gaffke, G., Wybranski, C.,
Mohnike, K., Wonneberger, U., Pech, M., Jung-
nickel, K., Ricke, J., and Strach, K. (2013). Mag-
netic resonance–guided freehand radiofrequency ab-
lation of malignant liver lesions: a new simplified and
time-efficient approach using an interactive open mag-
netic resonance scan platform and hepatocyte-specific
contrast agent. Invest Radiol, 48(6):422–428.
Fischer, S., Wickline, S., and Lorenz, C. (1999). Novel real-
time R-wave detection algorithm based on the vector-
cardiogram for accurate gated magnetic resonance ac-
quisitions. Magnet Reson Med, 42(2):361–370.
Haines, D. E., Beheiry, S., Akar, J. G., Baker, J. L., Bein-
born, D., Beshai, J. F., Brysiewicz, N., Chiu-Man, C.,
Collins, K. K., Dare, M., et al. (2014). Heart Rhythm
Society expert consensus statement on electrophysiol-
ogy laboratory standards: process, protocols, equip-
ment, personnel, and safety. Heart Rhythm, 11(8):e9–
e51.
Hunter, R. J., Jones, D. A., Boubertakh, R., Malcolme-
Lawes, L. C., Kanagaratnam, P., Juli, C. F., Davies,
D. W., Peters, N. S., Baker, V., Earley, M. J., et al.
(2013). Diagnostic accuracy of cardiac magnetic res-
onance imaging in the detection and characterization
of left atrial catheter ablation lesions: a multicenter
experience. J Cardiovasc Electrophysiol, 24(4):396–
403.
Kakareka, J. W., Faranesh, A. Z., Pursley, R. H., Campbell-
Washburn, A., Herzka, D. A., Rogers, T., Kanter, J.,
Ratnayaka, K., Lederman, R. J., and Pohida, T. J.
(2018). Physiological Recording in the MRI En-
vironment (PRiME): MRI-compatible hemodynamic
recording system. IEEE J Transl Eng Health Med.
Koopmann, M. and Marrouche, N. (2013). Why hesitate in-
troducing real-time magnetic resonance imaging into
the electrophysiological labs? Europace, 15(1):7–8.
Kreger, K. and Giordano, C. (1992). Biopotential adaptive
filtering in an MR environment. In Proceedings of the
SMRM 12th Annual Meeting, Berlin, volume 661.
Krug, J. (2015). Improved cardiac gating and patient mon-
itoring in high field magnetic resonance imaging by
means of electrocardiogram signal processing. Dis-
sertation, Otto-von-Guericke Universit
¨
at Magdeburg.
Krug, J., Rose, G., Clifford, G., and Oster, J. (2013). ECG-
Based Gating in Ultra High Field Cardiac MRI us-
ing an Independent Component Analysis Approach.
J Cardiovasc Magn Reson, 15(104):1–13.
Krug, J., Rose, G., Stucht, D., Clifford, G., and Oster, J.
(2012). Filtering the Magnetohydrodynamic Effect
from 12-lead ECG Signals using Independent Com-
ponent Analysis. In Proc IEEE Comput Cardiol,
Krakow, Poland.
Laudon, M. K., Webster, J. G., Frayne, R., and Grist, T. M.
(1998). Minimizing Interference from Magnetic Res-
onance Imagers During Electrocardiography. IEEE
Trans Biomed Eng, 45(2):160–164.
Progress of MRI-guided EP Interventions is Hampered by a Lack of ECG-based Patient Monitoring – An Engineering Perspective
207