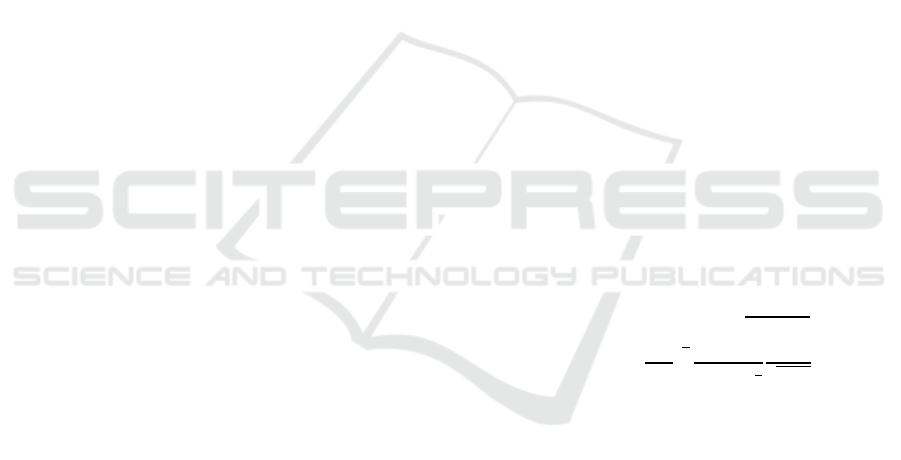
The purpose of this paper is to present a detailed
analysis of the three approaches for locating
exoplanets: radial velocity, transit and direct imaging.
In order to offer a analytically and systematically
sumamry of the current state of exoplanet detection
research as well as possible directions for future
development, this paper examines the guiding
principles, detector structures and current results of
these methods. The main part of the paper introduces
the three exploration methods in detail, including the
principle of each method, the structure of the probe
and the specific results in recent years. In addition,
the limitations of current planetary research and the
future prospects of this field are analysed.
2. DESCRIPTIONS OF PLANET
SEARCHING
Planet searching involves many aspects to be
analyzed and studied, and they provide important
information to further analyze the characteristics and
potential habitability of newfound planets. The
elements to be determined include the planetary
properties and the potential habitability of discovered
exoplanets. Planetary properties include radius, mass,
density and atmospheric composition of a planet.
Both radius and mass are measured at the first to
further obtain the density and atmospheric
composition. For the measurement of the radius,
scientists usually use transit method. When a planet
transits, it causes dimming of the star’s light. By
transit, the planet’s size relative to the star can be
determined. Regarding to the measurement of mass,
velocity method is used in most cases.
By knowing the planet’s mass and radius, the
density of the planet can be calculated using density
formula. Besides, the density can also provide the
information of an exoplanet’s composition. A planet's
atmosphere absorbs some starlight as it passes
through its star, which can be used to determine the
composition of the atmosphere. As for detailed
atmospheric condition, missions like the JWST will
provide strong support (Pepe, et al., 2020).
The factors to assess whether a planet could
support life include the planet's location within the
habitable zone, surface temperature, and geological
activity. With measurement of the star’s luminosity
and the planet’s distance from the star, whether its
location is within the habitable zone can be
determined. However, even if a planet’s location is
determined as the habitable zone, it doesn’t mean a
guarantee of habitability since it merely indicates the
signature of water.
Surface temperature can be affected by several
factors including the distance, its atmosphere, and its
reflectivity. For those planets with thick atmosphere,
closer distance and smaller reflectivity, their surface
temperature is greater; and vice versa (Shields, et al.,
2016). Geological activity is also very important to
maintain a planet’s habitability in the long run. It
generally includes two main factors: volcanism and
plate tectonics. They both contribute to producing and
recycling carbon and other elements, which is crucial
for climate stability and nutrient distribution.
3 RADIAL VELOCITY
Because of the planet's gravitational pull, a star with
planets orbiting it moves in a small orbit, making it
impossible for the star to remain stationary. This
movement is caused by the Doppler effect, which
periodically shifts the star's spectral lines. The star's
light spectrum has redshift when it moves away from
Earth and blueshift when it moves closer to the planet.
This observation forms the basis of the approach. The
existence of a planet and details about it, like its mass
and orbit, can be determined by measuring those
changes in the star's color lines (Ribas, et al., 2018;
Smith, et al., 2021).
The velocity change () can be calculated using
the Doppler formula:
(1)
(2)
where i is the inclination of the orbit, t is the time of
observation, t
0
is the time of periastron, and P is the
orbital period, G is the gravitational constant, M
p
and
M
*
are the mass of the planet and star, and e is the
eccentricity.
Detection using radial velocity measurements
basically depends on the spectrograph. There are
three important components of the spectrograph.
These fibers maintain a steady and uniform light
delivery while guiding starlight into
the spectrograph. In order to preserve the accuracy
required for radial velocity measurements, stabilized
optical fibers are used to reduce any possible signal
loss or distortion. Accurate measurements of radial
velocity require extremely stable wavelength
calibration. Reference light sources, such as Th-Ar
(thorium-argon) lamps and laser frequency combs,
are used to achieve this. To measure the star's
spectrum, these calibration systems provide a set of
IAMPA 2024 - International Conference on Innovations in Applied Mathematics, Physics and Astronomy
294