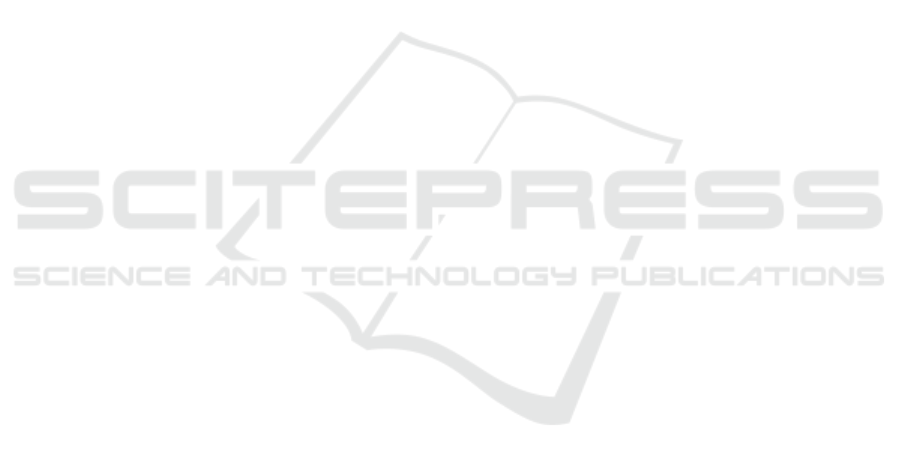
Franz, J., Menin, A., and Nedel, L. (2016). Lossless multi-
tasking: Using 3d gestures embedded in mouse devices.
In IEEE SVAR, pages 109–116. IEEE.
Froehlich, B., Hochstrate, J., Skuk, V., and Huckauf, A. (2006).
The globefish and the globemouse: Two new six degree
of freedom input devices for graphics applications. In
CHI ’06, pages 191–199. ACM Press.
Grossman, T. and Balakrishnan, R. (2005). The bubble cursor:
Enhancing target acquisition by dynamic resizing of the
cursor’s activation area. In CHI ’05, page 281–290.
ACM Press.
Hart, S. G. and Staveland, L. E. (1988). Development of
nasa-tlx (task load index): Results of empirical and
theoretical research. In Adv. Psychol., volume 52, pages
139–183. Elsevier.
Hinckley, K. and Sinclair, M. (1999). Touch-sensing input
devices. In CHI ’99, pages 223–230. ACM Press.
Hinckley, K., Sinclair, M., Hanson, E., Szeliski, R., and
Conway, M. (1999). The videomouse: A camera-based
multi-degree-of-freedom input device. In UIST ’99,
pages 103–112. ACM Press.
Hourcade, J. P., Crowther, M., and Hunt, L. (2007). Does
mouse size affect study and evaluation results? a study
comparing preschool children’s performance with small
and regular-sized mice. In IDC ’07, pages 109–116.
ACM Press.
Hutchings, D. R., Smith, G., Meyers, B., Czerwinski, M., and
Robertson, G. (2004). Display space usage and window
management operation comparisons between single
monitor and multiple monitor users. In AVI ’04, pages
32–39. ACM Press.
Kim, S., Lee, B., Van Gemert, T., and Oulasvirta, A. (2020).
Optimal sensor position for a computer mouse. In CHI
’20, pages 1–13. ACM Press.
Martin, B. and Raisamo, R. (2004). Trackmouse: A new
solution for 2+2d interactions. In NordiCHI ’04, pages
89–92. ACM Press.
McGuffin, M. and Balakrishnan, R. (2002). Acquisition of
expanding targets. In CHI ’02, pages 57–64. ACM.
Microsoft Corporation (2022). Microsoft arc mouse.
Mistry, P. and Maes, P. (2011). Mouseless: A computer mouse
as small as invisible. In CHI EA ’11, pages 1099–1104.
ACM Press.
Ortega, M. and Nigay, L. (2009). Airmouse: Finger gesture for
2d and 3d interaction. In Human-Computer Interaction –
INTERACT 2009, pages 214–227. Springer.
Perelman, G., Serrano, M., Raynal, M., Picard, C., Derras, M.,
and Dubois, E. (2015). The roly-poly mouse: Designing
a rolling input device unifying 2d and 3d interaction. In
CHI ’15, pages 327–336. ACM Press.
Peters, M. and Ivanoff, J. (1999). Performance asymmetries
in computer mouse control of right-handers, and left-
handers with left- and right-handed mouse experience.
J. Motor Behav., 31(1):86–94.
Roth, V. and Turner, T. (2009). Bezel swipe: Conflict-free
scrolling and multiple selection on mobile touch screen
devices. In CHI ’09, pages 1523–1526. ACM.
Saidi, H., Serrano, M., Irani, P., and Dubois, E. (2017).
Tdome: A touch-enabled 6dof interactive device for
multi-display environments. In CHI ’17, pages 5892–
5904. ACM Press.
Sato, K., Imada, S., Mazume, S., and Nakashima, M. (2020).
A mechanism of window switching prediction based
on user operation history to facilitate multitasking. In
Barolli, L., Nishino, H., Enokido, T., and Takizawa, M.,
editors, Adv. Networked-Based Inf. Syst., pages 154–166.
Springer.
Serrano, M., Lecolinet, E., and Guiard, Y. (2013). Bezel-tap
gestures: Quick activation of commands from sleep
mode on tablets. In CHI ’13, pages 3027–3036. ACM.
Shi, K., Subramanian, S., and Irani, P. (2009). Pressuremove:
Pressure input with mouse movement. In INTERACT
2009, pages 25–39. Springer.
Smith, G., Baudisch, P., Robertson, G., Czerwinski, M.,
Meyers, B., Robbins, D., and Andrews, D. (2003).
GroupBar: The TaskBar evolve. In OzCHI ’03, pages
34–43. ACM Press.
Son, S., Jung, J., Ham, A., and Lee, G. (2023). Touchwheel:
Enabling flick-and-stop interaction on the mouse wheel.
Int. J. Hum.-Comput. Inter., 0(0):1–13.
Tak, S., Cockburn, A., Humm, K., Ahlstr
¨
om, D., Gutwin,
C., and Scarr, J. (2009). Improving window switch-
ing interfaces. In INTERACT 2009, pages 187–200.
Springer.
Tak, S., Scarr, J., Gutwin, C., and Cockburn, A. (2011).
Supporting window switching with spatially consistent
thumbnail zones: Design and evaluation. In INTERACT
2011, pages 331–347. Springer.
Tashman, C. (2006). Windowscape: a task oriented window
manager. In UIST ’06, pages 77–80. ACM Press.
Tashman, C. and Edwards, W. K. (2012). Windowscape:
Lessons learned from a task-centric window manager.
ACM Trans. Comput.-Hum. Interact., 19(1):8:1–8:33.
Taylor, S., Keskin, C., Hilliges, O., Izadi, S., and Helmes,
J. (2014). Type–hover–swipe in 96 bytes: A motion
sensing mechanical keyboard. In CHI ’14, pages
1695–1704. ACM Press.
Ultraleap (2013). Leap motion controller.
Villar, N., Izadi, S., Rosenfeld, D., Benko, H., Helmes, J.,
Westhues, J., Hodges, S., Ofek, E., Butler, A., Cao, X.,
et al. (2009). Mouse 2.0: Multi-touch meets the mouse.
In UIST ’09, pages 33–42. ACM Press.
Warr, A., Chi, E. H., Harris, H., Kuscher, A., Chen, J.,
Flack, R., and Jitkoff, N. (2016). Window shopping: A
study of desktop window switching. In CHI ’16, pages
3335–3338. ACM Press.
Xu, Q. and Casiez, G. (2010). Push-and-pull switching:
Window switching based on window overlapping. In
CHI ’10, pages 1335–1338. ACM Press.
Yang, X.-D., Mak, E., McCallum, D., Irani, P., Cao, X., and
Izadi, S. (2010). Lensmouse: Augmenting the mouse
with an interactive touch display. In CHI ’10, pages
2431–2440. ACM Press.
Zeidler, C., Lutteroth, C., and Weber, G. (2013). An evaluation
of stacking and tiling features within the traditional
desktop metaphor. In Kotz
´
e, P., Marsden, G., Lindgaard,
G., Wesson, J., and Winckler, M., editors, INTERACT
2013, pages 702–719. Springer.
HUCAPP 2025 - 9th International Conference on Human Computer Interaction Theory and Applications
560