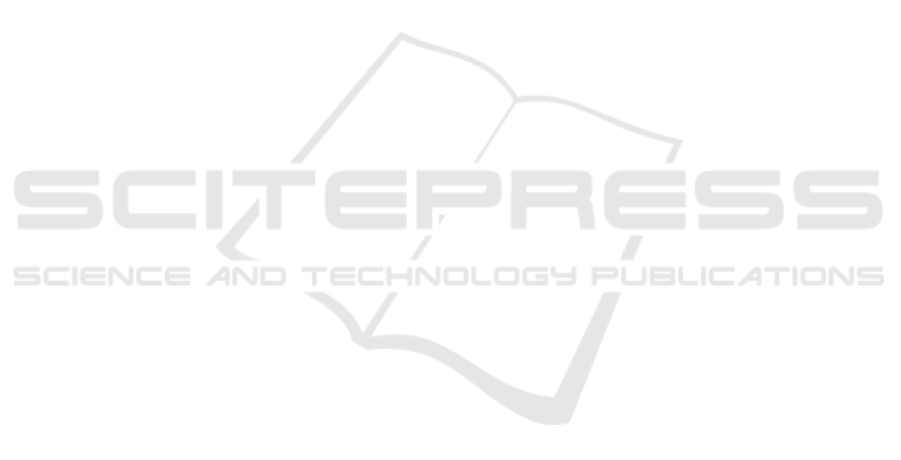
ogy – CRYPTO 2004, pages 56–72, Berlin, Heidel-
berg. Springer Berlin Heidelberg.
Chaum, D. (1985). Security without identification: Trans-
action systems to make big brother obsolete. Com-
mun. ACM, 28(10):1030–1044.
Chen, Y., Lombardi, A., Ma, F., and Quach, W. (2021).
Does fiat-shamir require a cryptographic hash func-
tion? In Malkin, T. and Peikert, C., editors, Ad-
vances in Cryptology – CRYPTO 2021, pages 334–
363, Cham. Springer International Publishing.
European Commission (2016). Regulation (EU) 2016/679
of the European Parliament and of the Council of 27
April 2016 on the protection of natural persons with
regard to the processing of personal data and on the
free movement of such data, and repealing Directive
95/46/EC (General Data Protection Regulation) (Text
with EEA relevance).
European Telecommunications Standards Institute (2018).
ETSI TS 123 501 V15.3.0: 5G; System Architecture
for the 5G System. https://www.etsi.org/standards.
Accessed: 2023-08-25.
F
¨
orster, D., Kargl, F., and L
¨
ohr, H. (2014). Puca: A
pseudonym scheme with user-controlled anonymity
for vehicular ad-hoc networks (vanet). In 2014 IEEE
Vehicular Networking Conference (VNC), pages 25–
32.
Galego, N. M. C. and Pascoal, R. M. (2022). Cybersecurity
in smart cities: Technology and data security in intel-
ligent transport systems. In Mesquita, A., Abreu, A.,
and Carvalho, J. V., editors, Perspectives and Trends
in Education and Technology, pages 17–33, Singa-
pore. Springer Singapore.
Hammi, B., Monteuuis, J.-P., and Petit, J. (2022). Pkis in
c-its: Security functions, architectures and projects: A
survey. Vehicular Communications, 38:100531.
Heng, X., Qin, S., Xiao, Y., Wang, J., Tao, Y., and Zhang,
R. (2022). A strong secure v2i authentication scheme
from pki and accumulator. In 2022 2nd International
Conference on Consumer Electronics and Computer
Engineering (ICCECE), pages 98–103.
Kakvi, S. A., Martin, K. M., Putman, C., and Quaglia,
E. A. (2023). Sok: Anonymous credentials. In
G
¨
unther, F. and Hesse, J., editors, Security Standardi-
sation Research, pages 129–151, Cham. Springer Na-
ture Switzerland.
Khan, A., Ahmad, A., Ahmed, M., Sessa, J., and Anisetti,
M. (2022). Authorization schemes for internet
of things: requirements, weaknesses, future chal-
lenges and trends. Complex & Intelligent Systems,
8(5):3919–3941.
Lauinger, J., Ernstberger, J., Regnath, E., Hamad, M., and
Steinhorst, S. (2021). A-poa: Anonymous proof of
authorization for decentralized identity management.
In 2021 IEEE International Conference on Blockchain
and Cryptocurrency (ICBC), pages 1–9.
Liao, D., Li, H., Sun, G., Zhang, M., and Chang, V. (2018).
Location and trajectory privacy preservation in 5g-
enabled vehicle social network services. Journal of
Network and Computer Applications, 110:108–118.
Mitsunari, S. (2019). ”MCL cryptolibrary”. https://github.
com/herumi/mcl.
Ren, Y., Liu, X., Wu, Q., Wang, L., Zhang, W., et al. (2022).
Cryptographic accumulator and its application: A sur-
vey. Security and Communication Networks, 2022.
Salin, H. (2023a). Acca: A decentralized and accumulator-
based authentication and authorization architecture
for autonomous iot in connected infrastructures. In
Wills, G. B., Butty
´
an, L., Kacsuk, P., and Chang, V.,
editors, Proceedings of the 8th International Confer-
ence on Internet of Things, Big Data and Security,
IoTBDS 2023, Prague, Czech Republic, April 21-23,
2023, pages 170–177. SCITEPRESS.
Salin, H. (2023b). Pseudonym swapping with secure accu-
mulators and double diffie-hellman rounds in coopera-
tive intelligent transport systems. In Kallel, S., Jmaiel,
M., Zulkernine, M., Hadj Kacem, A., Cuppens, F., and
Cuppens, N., editors, Risks and Security of Internet
and Systems, pages 223–238, Cham. Springer Nature
Switzerland.
Salin, H., Fokin, D., and Johansson, A. (2021). Authen-
ticated multi-proxy accumulation schemes for dele-
gated membership proofs. In Luo, B., Mosbah, M.,
Cuppens, F., Othmane, L. B., Cuppens, N., and Kallel,
S., editors, Risks and Security of Internet and Systems
- 16th International Conference, CRiSIS 2021, Virtual
Event, Ames, USA, November 12-13, 2021, Revised
Selected Papers, volume 13204 of Lecture Notes in
Computer Science, pages 162–171. Springer.
Shahab, S., Agarwal, P., Mufti, T., and Obaid, A. J. (2022).
Siot (social internet of things): A review. In Fong, S.,
Dey, N., and Joshi, A., editors, ICT Analysis and Ap-
plications, pages 289–297, Singapore. Springer Na-
ture Singapore.
Sharma, A., Pilli, E. S., Mazumdar, A. P., and Gera, P.
(2020). Towards trustworthy internet of things: A sur-
vey on trust management applications and schemes.
Computer Communications, 160:475–493.
Valle, F., Cooney, M., Mikhaylov, K., and Vinel, A. (2021).
The integration of uavs to the c-its stack. In 2021 IEEE
29th International Conference on Network Protocols
(ICNP), pages 1–6.
Zeddini, B., Maachaoui, M., and Inedjaren, Y. (2022). Se-
curity threats in intelligent transportation systems and
their risk levels. Risks, 10(5).
Zuo, X., Li, L., Luo, S., Peng, H., Yang, Y., and Gong,
L. (2021). Privacy-preserving verifiable graph inter-
section scheme with cryptographic accumulators in
social networks. IEEE Internet of Things Journal,
8(6):4590–4603.
IoTBDS 2025 - 10th International Conference on Internet of Things, Big Data and Security
288